Local Anesthetics: Clinical Pharmacology and Rational Selection
Jeff Gadsden
Local anesthetics (LAs) prevent or relieve pain by interrupting nerve conduction. They bind to specific receptor sites on the sodium (Na+) channels in nerves and block the movement of ions through these pores. Both the chemical and pharmacologic properties of individual LA drugs determine their clinical properties. This chapter discusses the basics of the mechanism of action of LAs, their clinical use, and systemic toxicity prevention and treatment.
Nerve Conduction
Nerve conduction involves the propagation of an electrical signal generated by the rapid movement of small amounts of several ions (Na+ and potassium K+) across a nerve cell membrane. The ionic gradient for Na+ (high extracellularly and low intracellularly) and K+ (high intracellularly and low extracellularly) is maintained by a Na+-K+ pump mechanism within the nerve. In the resting state, the nerve membrane is more permeable to K+ ions than to Na+ ions, resulting in the continuous leakage of K+ ions out of the interior of the nerve cell. This leakage of cations, in turn, creates a negatively charged interior relative to the exterior, resulting in an electric potential of 60–70 mV across the nerve membrane.
Receptors at the distal ends of sensory nerves serve as sensors and transducers of various mechanical, chemical, or thermal stimuli. Such stimuli are converted into minuscule electric currents. For example, chemical mediators released with a surgical incision react with these receptors and generate small electric currents. As a result, the electric potential across a nerve membrane near the receptor is altered, making it less negative. If the threshold potential is achieved, an action potential results, with a sudden increase in the permeability of the nerve membrane to Na+ ions and a resultant rapid influx of positively charged Na+ ions. This causes a transient reversal of charge, or depolarization. Depolarization generates a current that sequentially depolarizes the adjacent segment of the nerve, thus “activating” the nerve and sending a wave of sequential polarization down the nerve membrane.
Repolarization takes place when sodium permeability decreases and K+ permeability increases, resulting in an efflux of K+ from within the cell and restoration of the electrical balance. Subsequently, both ions are restored to their initial intracellular and extracellular concentrations by the Na+-K+-adenosine triphosphate pump mechanism. Because the rapid influx of Na+ ions occurs in response to a change in the transmembrane potential, Na+ channels in the nerve are characterized as “voltage gated”. These channels are protein structures with three subunits that penetrate the full depth of the membrane bilayer and are in communication with both the extracellular surface of the nerve membrane and the axoplasm (interior) of the nerve. LAs prevent the generation and conduction of nerve impulses by binding to the α subunit of the Na+ channel and preventing the influx of Na+ into the cell, halting the transmission of the advancing wave of depolarization down the length of the nerve.
A resting nerve is less sensitive to a LA than a nerve that is repeatedly stimulated. A higher frequency of stimulation and a more positive membrane potential cause a greater degree of transmission block. These frequency- and voltage- dependent effects of LAs occur because repeated depolarization increases the chance that a LA molecule will encounter a Na+ channel that is in the activated, or open, form—as opposed to the resting form—which has a much greater affinity for LA. In general, the rate of dissociation from the receptor site in the pore of the Na+ channel is critical for the frequency dependence of LA action.
Structure–Activity Relationship of Local Anesthetics
The typical structure of a LA consists of hydrophilic and hydrophobic domains separated by an intermediate ester or amide linkage. The hydrophilic group is usually a tertiary amine, and the hydrophobic domain is an aromatic moiety. The nature of the linking group determines the pharmacologic properties of LA agents. The physicochemical properties of these agents largely influence their potency and duration of action. For instance, greater lipid solubility increases both the potency and duration of their action. This is due to a greater affinity of the drug to lipid membranes and therefore greater proximity to its sites of action. The longer the drug remains in the vicinity of the membrane, rather than being replaced by the blood, the more likely the drug will be to effect its action on the Na+ channel in the membrane. Unfortunately, greater lipid solubility also increases toxicity, decreasing the therapeutic index for more hydrophobic drugs.
The pKa (the pH at which 50% of the drug is ionized and 50% is present as base) of the LA is related to pH and the concentrations of the cationic and base forms by the Henderson-Hasselbalch equation: .
The pKa generally correlates with the speed of onset of action of most amide LA drugs; the closer the pKa to the body pH, the faster the onset. The coexistence of the two forms of the drug—the charged cation and the uncharged base—is important because drug penetration of the nerve membrane by the LA requires the base (unionized) form to pass through the nerve lipid membrane; once in the axoplasm of the nerve, the base form can accept a hydrogen ion and equilibrate into the cationic form. The cationic form is predominant and produces a blockade of the Na+ channel. The amount of base form that can be in solution is limited by its aqueous solubility.
An ester or an amide linkage is present between the lipophilic end (benzene ring) and the hydrophilic end (amino group) of the molecule. The type of linkage determines the site of metabolic degradation of the drug. Ester-linked LAs are metabolized in plasma by pseudocholinesterase, whereas amide-linked drugs undergo metabolism in the liver.
The Onset and Duration of Blockade
Local Anesthetic Diffusion
A mixed peripheral nerve or nerve trunk consists of individual nerves surrounded by an investing epineurium. When a LA is deposited in proximity to a peripheral nerve, it diffuses from the outer surface toward the core along a concentration gradient. Consequently, nerves located in the outer mantle of the mixed nerve are blocked first. These fibers are usually distributed to more proximal anatomic structures than those situated near the core of the mixed nerve and often are motor fibers. When the volume and concentration of LA solution deposited in the vicinity of the nerve are adequate, the LA eventually diffuses inward to block the more centrally located fibers. In this way, the block evolves from proximal structures to distal structures. Smaller amounts and concentrations of a drug only block the nerves in the mantle and smaller and more sensitive central fibers.
Onset of Blockade
In general, LAs are deposited as close to the nerve as possible, preferably into the tissue sheaths (e.g., brachial plexus, lumbar plexus) or epineurial sheaths of the nerves (e.g., femoral, sciatic). The actual site of local anesthetic injection and its relationship to the nerve structures is much better understood since the advent of the use of ultrasound guidance during nerve blockade. Intraneural or sub-epineural injections reportedly occur relatively frequently with some peripheral nerve blocks. The available data indicate that such injections result in faster onset of blockade, most likely due to the intimate proximity of LA to the nerve tissue. This is hardly surprising because the LA must diffuse from the site of injection to the nerve, the site of action. However, intraneural injections should not be recommended as a safe practice despite limited reports suggesting that intraneural injections do not inevitably lead to nerve injury. These data must be interpreted with caution because the term intraneural injection is often used loosely to denote injections within epineurium or even tissue sheaths that envelop the peripheral nerves or plexi. However, neurologic injury is much more likely to occur should an intraneural injection occur intrafascicularly.
The rate of diffusion across the nerve sheath is determined by the concentration of the drug, its degree of ionization (ionized LA diffuses more slowly), its hydrophobicity, and the physical characteristics of the tissue surrounding the nerve.
Duration of Blockade
The duration of nerve block anesthesia depends on the physical characteristics of the LA and the presence or absence of vasoconstrictors. The most important physical characteristic is lipid solubility. In general, LAs can be divided into three categories: short acting (e.g., 2-chloroprocaine, 45–90 minutes), intermediate duration (e.g., lidocaine, mepivacaine, 90–180 minutes), and long acting (e.g., bupivacaine, levobupivacaine, ropivacaine, 4–18 hours). The degree of block prolongation with the addition of a vasoconstrictor appears to be related to the intrinsic vasodilatory properties of the LA; the more intrinsic vasodilatory action the LA has, the more prolongation is achieved with addition of a vasoconstrictor.
Although this discussion is in line with current clinical teaching, it is really more theoretical than of significant clinical relevance. For instance, dense blocks of the brachial plexus with 2-chloroprocaine are likely to outlast weak poor quality blocks with bupivacaine. In addition, classical teaching does not take into account the nerve to be anesthetized. As an example, a sciatic nerve block with bupivacaine lasts almost twice as long as an interscalene or lumbar plexus block with the same drug dose and concentration. These differences must be kept in mind to time and predict the resolution of blockade properly.
Differential Sensitivity of Nerve Fibers to Local Anesthetics
Two general rules apply regarding susceptibility of nerve fibers to LAs: First, smaller nerve fibers are more susceptible to the action of LAs than large fibers (Figure 2-1). Smaller fibers are preferentially blocked because a shorter length of axon is required to be blocked to halt the conduction completely. Second, myelinated fibers are more easily blocked than nonmyelinated fibers because local anesthetic pools near the axonal membrane. This is why C-fibers, which have a small diameter (but are unmyelinated), are the most resistant fibers to LA.
FIGURE 2-1. Differential rate of nerve blockade.
The sensitivity of a fiber to LAs is not determined by whether it is sensory or motor. In fact, muscle proprioceptive afferent (A-beta) and motor efferent fibers (A-alpha) are equally sensitive. These two types of fibers have the same diameter, which is larger than that of the A-gamma fibers that supply the muscle spindles. It is the more rapid blockade of these smaller A-gamma fibers, rather than of the sensory fibers, that leads to the preferential loss of muscle reflexes. Similarly, in large nerve trunks, motor fibers are often located in the outer portion of the bundle and are more accessible to LA. Thus motor fibers may be blocked before sensory fibers in large mixed nerves.
The differential rate of blockade exhibited by fibers of varying sizes and firing rates is of considerable practical importance. Fortunately, the sensation of pain is usually the first modality to disappear; it is followed by the loss of sensations of cold, warmth, touch, deep pressure, and, finally, loss of motor function, although variation among patients and different nerves is considerable.
Local Anesthetics and pH
LA drugs, as previously described, pass through the nerve membrane in a nonionized lipid-soluble base form; when they are within the nerve axoplasm, they must equilibrate into an ionic form to be active within the Na+ channel. The rate-limiting step in this cascade is penetration of the LA through the nerve membrane. LAs are unprotonated amines and as such tend to be relatively insoluble (Figure 2-2). For this reason, they are manufactured as water-soluble salts, usually hydrochlorides. Although LAs are weak bases (typical pKa values range from 7.5–9), their hydrochloride salts are mildly acidic. This property increases the stability of LA esters and any accompanying vasoconstrictor substance. However, this means that the cationic form predominates in solution.
FIGURE 2-2. Local anesthetics and pH. Local anesthetics pass through the nerve membrane in a nonionized, lipid-soluble base form. When they are within the nerve axoplasm, they must equilibrate into an ionic form to exert their action on the Na+ channel.
For this reason, sodium bicarbonate (NaHCO3) is often added to LA. This increases the amount of drug in the base form, which slightly shortens the onset time. Obviously, the limiting factor for pH adjustment is the solubility of the base form of the drug. Unfortunately, only small changes in pH can be achieved by the addition of bicarbonate because of the limited solubility of the base. As such, only small decreases in onset time are realized. For instance, with the alkalinization of bupivacaine, an increase in the amount of base in solution is limited by the minimal solubility of free base in solution. For each LA, there is a pH at which the amount of base in solution is maximal (a saturated solution). Further increases in pH result in precipitation of the drug and do not produce an additional shortening of onset time.
Protein Binding
LAs are in large part bound to plasma and tissue proteins. However, they are pharmacologically active only in the free, unbound state. The most important binding proteins for LAs in plasma are albumin and alpha1-acid glycoprotein (AAG). The binding to AAG is characterized as high-affinity but low-capacity binding; hence LAs bind to AAG preferentially compared with albumin. However, binding to AAG is easily saturated with clinically achieved blood levels of LA. Once AAG saturation occurs, any additional binding is to albumin. Albumin can bind LA drugs in plasma in concentrations many times greater than those clinically achieved.
Note that the fraction of drugs bound to protein in plasma correlates with the duration of LA activity: bupivacaine > etidocaine > ropivacaine > mepivacaine > lidocaine > procaine and 2-chloroprocaine. However, no direct relationship exists between LA plasma protein binding and binding to specific membrane-bound Na+ channels. Rather, there is a direct correlation between protein binding and lipid solubility, as there is for all drugs. The more lipid soluble the drug, the more likely it will remain in the lipid-rich environment of the axonal membrane where the Na+ channel resides.
The degree of protein binding of a particular LA is concentration dependent and influenced by the pH of the plasma. The percentage of drug bound decreases as the pH decreases. This is important because with the development of acidosis, as may occur with LA-induced seizures or cardiac arrest, the amount of free drug increases. The magnitude of this phenomenon varies among LAs, and it is much more pronounced with bupivacaine than with lidocaine. For instance, as the binding decreases from 95% to 70% with acidosis, the amount of free bupivacaine increases from 5% to 30% (a factor of 6), although the total drug concentration remains unchanged. Because of this increase in free drug, acidosis renders bupivacaine markedly more toxic.
Systemic Toxicity of Local Anesthetics
In addition to interrupting peripheral nerve conduction, LAs interfere with the function of all organs in which the conduction or transmission of nerve impulses occurs. For instance, they have important effects on the central nervous system (CNS), the autonomic ganglia, the neuromuscular junction, and musculature. The risk of such adverse reactions is proportional to the concentration of LA achieved in the circulation.
Plasma Concentration of Local Anesthetics
The following factors determine the plasma concentration of LAs:
• The dose of the drug administered
• The rate of absorption of the drug
• Site injected, vasoactivity of the drug, use of vasoconstrictors
• Biotransformation and elimination of the drug from the circulation
Noted that although the peak level of a LA is directly related to the dose administered, administration of the same dose at different sites results in marked differences in peak blood levels. This explains why large doses of LA can be used with peripheral nerve blocks without the toxicity that would be seen with an intramuscular or intravenous (IV) injection. It is also for this reason that the adherence to strictly defined maximum doses of LAs is nonsensical. As an example, 150 mg of ropivacaine at the popliteal fossa will result in a markedly different plasma level than the same dose administered intercostally. Careful consideration of patient factors and the requirements of the block should precede selection of LA type and dose for peripheral nerve block.
Short-acting ester local anesthetics are inherently safer with respect to systemic toxicity due to their clearance by pseudocholinesterase. In the case of 2-chloroprocaine, peak blood levels achieved are affected by the rate at which the LA drug undergoes biotransformation and elimination (plasma half-life of about 45 seconds–1 minute). In contrast, peak blood levels of amide-linked LA drugs are primarily the result of absorption.
Central Nervous System Toxicity
The symptoms of CNS toxicity associated with LAs are a function of their plasma level (Figure 2-3). Toxicity is typically first expressed as stimulation of the CNS, producing restlessness, disorientation, and tremor. As the plasma concentration of the LA increases, tonic-clonic seizures occur; the more potent the LA, the more readily convulsions may be produced. With even higher levels of LA, central stimulation is followed by depression and respiratory failure, culminating in coma.
FIGURE 2-3. Progression of local anesthetic toxicity.
The apparent stimulation and subsequent depression produced by applying LAs to the CNS presumably are due to depression of neuronal activity. Selective depression of inhibitory neurons is thought to account for the excitatory phase in vivo. However, rapid systemic administration of a LA may produce death with no, or only transient, signs of CNS stimulation. Under these conditions, the concentration of the drug probably rises so rapidly that all neurons are depressed simultaneously. Airway control and support of respiration constitute essential treatment. Benzodiazepines or a small dose of propofol (e.g., 0.5–1.0 mg/kg) administered IV in small doses are the drugs of choice for aborting convulsions. The use of benzodiazepines as premedication is often recommended to elevate the seizure threshold; however, they must be used with caution because respiratory depression with excessive sedation may produce respiratory acidosis with a consequent higher level of free drug in the serum.
Cardiovascular Toxicity
The primary site of action of LAs in the cardiovascular system is the myocardium, where they decrease electrical excitability, conduction rate, and the force of myocardial contraction. Most LAs also cause arteriolar dilatation, contributing to hypotension. Cardiovascular effects typically occur at higher systemic concentrations than those at which effects on the CNS are produced. However, note that it is possible for cardiovascular collapse and death to occur even in an absence of the warning signs and symptoms of CNS toxicity. It is believed that this is probably the result of action on the pacemaker cells or the sudden onset of ventricular fibrillation. In animal studies on LA cardiotoxicity, all caused dose-dependent depression of the contractility of cardiac muscle. This depressant effect on cardiac contractility parallels the anesthetic potency of the LA in blocking nerves. Therefore, bupivacaine, which is four times more potent than lidocaine in blocking nerves, is also four times more potent in depressing cardiac contractility. Deaths caused by a bupivacaine overdose have been associated with progressive prolongation of ventricular conduction and widening of the QRS complex, followed by the sudden onset of arrhythmia such as ventricular fibrillation.
Pregnancy and Local Anesthetic Toxicity
Plasma concentrations of AAG are also decreased in pregnant women and in newborns. This lowered concentration effectively increases the free fraction of bupivacaine in plasma, and it may have been an important contributing factor to the bupivacaine toxicity in pregnant patients and to the number of cardiac arrests that have been reported with inadvertent overdoses of bupivacaine in pregnant women. However, with intermediate-duration LAs (e.g., lidocaine and mepivacaine), smaller changes in protein binding occur during pregnancy, and the use of these LAs is not associated with an increased risk of cardiac toxicity during pregnancy.
Pharmacodynamics and Treatment of Local Anesthetic Toxicity
Blood levels of lidocaine associated with the onset of seizures appear to be in the range of 10 to 12 μg/mL. At these concentrations, inhibitory pathways in the brain are selectively disabled, and excitatory neurons can function unopposed. As the blood levels of lidocaine are increased further, respiratory depression becomes significant and at much higher levels (20–25 μg/mL), cardiotoxicity is manifested. In contrast, for bupivacaine, blood levels of approximately 4 μg/mL result in seizures, and blood levels between 4 and 6 μg/mL are associated with cardiac toxicity. This is reflective of a much lower therapeutic index for bupivacaine compared with lidocaine in terms of cardiac toxicity. In the setting of neurotoxicity without cardiac effects, high levels of LA in the brain rapidly dissipate and are redistributed to other tissue compartments. However, with the onset of significant cardiotoxicity, cardiac output diminishes, resulting in impairment in redistribution.
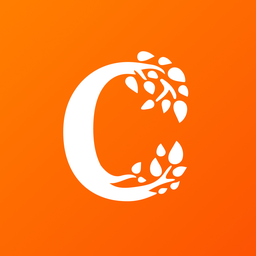
Full access? Get Clinical Tree
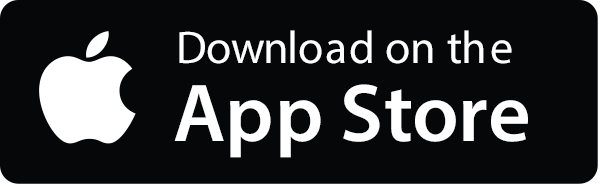
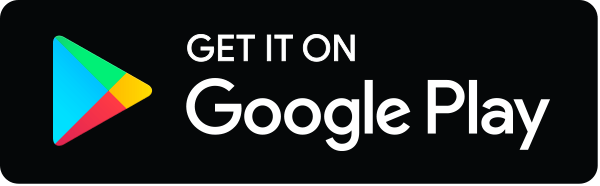