Key Points
- ▪
Local anesthetics block voltage-gated sodium channels and thereby interrupt initiation and propagation of impulses in axons, but they have a wide variety of other biologic actions, desirable and undesirable.
- ▪
Currently available local anesthetics are of two chemical classes: aminoesters and aminoamides.
- ▪
The low potency and lack of specificity of available local anesthetics are due in part to the very weak structural constraints at their binding site on the sodium channel. Most of their features derive from the requirement for high solubility, rapidly reversible protonation, and rapid diffusion in both aqueous environments and in the lipid phases of biologic membranes.
- ▪
Reversible protonation of the tertiary amine group tends to make local anesthetics less charged at more basic pH and more charged at neutral or acidic pH; the neutral base forms are more soluble in lipid environments, whereas the charged acid forms, the more potent species, are more soluble in aqueous environments.
- ▪
Aminoesters are metabolized primarily by plasma esterases, and aminoamides are metabolized primarily by hepatic cytochrome P450-linked enzymes.
- ▪
The principal systemic toxicities of local anesthetics involve the heart (including atrioventricular conduction block, arrhythmias, myocardial depression, and cardiac arrest) and the brain (including agitation, lethargy, seizures, and generalized central nervous system depression). Hypoxemia and acidosis exacerbate these toxicities. Resuscitation after bupivacaine overdose is particularly difficult. Therefore prevention of intravascular injection or overdose is crucial, and major nerve blockade should involve incremental, fractionated dosing. Ultrasound can reduce the incidence of systemic toxicity and Intralipid can help in the resuscitation.
- ▪
Local anesthetics are directly toxic to nerve at the concentrations supplied in commercial solutions. Intraneural concentrations during regional anesthesia are generally (but not always) below a threshold for toxicity because of spread of solutions through tissues and diffusion gradients from injection sites into nerve. Injection into a constrained tissue space increases the risk for local toxicity.
- ▪
Optimal use of local anesthetics in regional anesthesia requires an understanding of (1) the individual patient’s clinical situation; (2) the location, intensity, and duration of regional anesthesia and analgesia required; (3) anatomic factors affecting deposition of drug near nerves; (4) proper drug selection and dosing; and (5) ongoing assessment of clinical effects after administration of a local anesthetic.
- ▪
Single-stereoisomer (as opposed to a racemic mixture) formulations have been developed in an effort to reduce systemic toxicity and improve sensory selectivity but true sensory selectivity has not been achieved with drugs currently available.
- ▪
The most important research avenues to improve local anesthetic action are slow release formulations, targeting of specific sodium channel subtypes, and targeting of nociceptive fibers.
Acknowledgment
The editors, publisher, Dr. Charles B. Berde, and Dr. Philipp Lirk would like to thank Dr. Gary Strichartz for his contribution to this chapter in the prior edition of this work. It has served as the foundation for the current chapter.
Local anesthesia results from the blockade of nerve impulses to abolish sensation. All currently available, clinically useful agents are either aminoesters or aminoamides. These drugs, when applied in sufficient concentration at the site of action, prevent conduction of electrical impulses by the membranes of nerve and muscle. In addition to blockade of impulses, local anesthetics can inhibit various receptors, enhance release of glutamate, and depress the activity of certain intracellular signaling pathways. When local anesthetic agents are given systemically, the functions of cardiac, skeletal, and smooth muscle, as well as transmission of impulses in the central and peripheral nervous systems and within the specialized conducting system of the heart, may all be altered. Local anesthetics may abolish sensation in various parts of the body by topical application, injection near peripheral nerve endings and major nerve trunks, or instillation within the epidural or subarachnoid spaces. Toxicity may be local or systemic. The central nervous and cardiovascular systems are most commonly involved in acute clinical toxicity.
Basic Pharmacology
Chemistry
The Local Anesthetic Molecule
The typical local anesthetic molecule, exemplified by lidocaine and procaine ( Fig. 29.1 ), contains a tertiary amine attached to a substituted aromatic ring by an intermediate chain that almost always contains either an ester (see Fig. 29.1 ) or an amide linkage ( Fig. 29.2 ).


Local anesthetics may therefore be classified as aminoester or aminoamide compounds. The aromatic ring system gives a lipophilic (membrane-liking) character to its portion of the molecule, whereas the tertiary amine end is relatively hydrophilic, particularly since it is partially protonated and thus bears some positive charge in the physiologic pH range (see Fig. 29.2 ). The structures of commonly administered local anesthetics are given in Table 29.1 and their physicochemical properties in Table 29.2 .
Drug | Relative Conduction-Blocking Potency ∗ | Physiochemical Properties | |
---|---|---|---|
p K a † | Hydrophobicity † | ||
Low Potency | |||
Procaine | 1 | 8.9 | 100 |
Intermediate Potency | |||
Mepivacaine | 1.5 | 7.7 | 136 |
Prilocaine | 1.8 | 8.0 ‡ | 129 |
Chloroprocaine | 3 | 9.1 | 810 |
Lidocaine | 2 | 7.8 | 366 |
High Potency | |||
Tetracaine | 8 | 8.4 | 5822 |
Bupivacaine | 8 | 8.1 | 3420 |
Etidocaine | 8 | 7.9 | 7320 |
∗ Data derived from C fibers of isolated rabbit vagus and sciatic nerve.
† p K a and hydrophobicity at 36°C; hydrophobicity equals the octanol/buffer partition coefficient of the base. Values are ratios of concentrations.
Structure-Activity Relationships and Physicochemical Properties
The intrinsic potency and duration of action of local anesthetics are clearly dependent on certain features of the molecule.
Lipophilic-Hydrophilic Balance
The lipophilic versus hydrophilic character of a local anesthetic depends on the size of alkyl substituents on or near the tertiary amine and on the aromatic ring. “Lipophilicity” expresses the tendency of a compound to associate with membrane lipids, a property usually approximated by equilibrium partitioning into a hydrophobic solvent such as octanol. Although octanol-buffer partition coefficients are comparable to membrane-buffer partition coefficients for the uncharged species of local anesthetics, they severely underestimate membrane partitioning for the charged, protonated species, octanol being a poor model for the polar regions near the membrane surface where local anesthetics are concentrated. Here we use the term “hydrophobicity,” expressed as octanol-buffer partitioning, to describe a physicochemical property of local anesthetics.
Compounds with a more hydrophobic nature are obtained by increasing the size of the alkyl substituents. These agents are more potent and produce longer-lasting blocks than their less hydrophobic congeners do. For example, etidocaine, which has three more carbon atoms in the amine end of the molecule than lidocaine, is four times as potent and five times as long lasting when compared in the isolated sciatic nerve.
Hydrogen Ion Concentration
Local anesthetics in solution exist in a very rapid chemical equilibrium between the basic uncharged form (B) and the charged cationic form (BH + ). At a certain hydrogen ion concentration (log 10 −1 [−pH]) specific for each drug, the concentration of local anesthetic base in solution is equal to the concentration of charged cation. The logarithm of this hydrogen ion concentration is called p K a . The relationship is defined by
[BH+][B]=10pKa−pH
p K a values of local anesthetic agents in aqueous solution are listed in Table 29.2 . The tendency to be protonated also depends on environmental factors, such as temperature and ionic strength, and on the medium surrounding the drug. In the relatively apolar milieu of a membrane, the average p K a of local anesthetics is lower than in solution. This is chemically equivalent to saying that the membrane concentrates the base form of the local anesthetic more than it concentrates the protonated cation form. The pH of the medium containing the local anesthetic influences drug activity by altering the relative percentage of the base and protonated forms. For example, in inflamed tissues the pH is lower than normal, and local anesthetics are more protonated than in normal tissue and thus penetrate the tissue relatively poorly (see later). The relationship between p K a and the percentage of local anesthetic present in the cationic form is shown in Fig. 29.2 . As described later, there are dual effects of pH on clinical effectiveness, depending on where the local anesthetic is injected and the importance of the base form for tissue penetration.
Anatomy of the Peripheral Nerve
Each peripheral nerve axon possesses its own cell membrane, the axolemma. Nonmyelinated nerves, such as autonomic postganglionic efferent and nociceptive afferent C fibers, contain many axons encased in a single Schwann cell sheath. In contrast, all large motor and sensory fibers are enclosed in many layers of myelin, which consists of the plasma membranes of specialized Schwann cells that wrap themselves around the axon during axonal outgrowth. Myelin greatly increases the speed of nerve conduction by insulating the axolemma from the surrounding conducting salt medium and forcing the “action current” generated by an impulse to flow through the axoplasm to the nodes of Ranvier, which are periodic interruptions in the myelin sheath where the active impulse is regenerated ( Fig. 29.3 ). The Na + channels that serve generation and propagation of impulses are highly concentrated at the nodes of Ranvier of myelinated fibers but are distributed all along the axon of nonmyelinated fibers (see Fig. 29.3 ). A classification of peripheral nerves according to fiber size and physiologic properties is presented in Table 29.3 . It is important to note that different fiber classes are not only distinguished by diameter and myelin thickness, but also by the structure of the neuronal membrane and ion channel composition.

Fiber Class | Subclass | Myelin | Diameter (μm) | Conduction Velocity (m/sec) | Location | Function | Susceptibility to Local Anesthetic Block |
---|---|---|---|---|---|---|---|
A | αβδ | ++++ | 6-226-223-61-4 | 30-12030-12015-355-25 | Efferent to musclesAfferent from skin and jointsEfferent to muscle spindlesAfferent sensory nerves | MotorTactile, proprioceptionMuscle tonePain, cold temperature, touch | +++++++++++ |
B | + | <3 | 3-15 | Preganglionic sympathetic | Various autonomic functions | ++ | |
C | sC | − | 0.3-1.3 | 0.7-1.3 | Postganglionic sympathetic | Various autonomic functions | ++ |
dC | − | 0.4-1.2 | 0.1-2.0 | Afferent sensory nerves | Various autonomic functions Pain, warm temperature, touch | + |
Each axon has its own connective tissue covering, the endoneurium . A typical peripheral nerve consists of several axon bundles, or fascicles. Each fascicle of many axons is encased by a second connective tissue layer, the epithelial-like perineurium , and the entire nerve is wrapped in a loose outer sheath called the epineurium ( Fig. 29.4 ). To reach the nerve axon, a local anesthetic molecule must traverse any structures surrounding the nerve, such as the paraneurium of the distal sciatic nerve, the epineurium, the perineurium, and the endoneurium, as well as the neuronal plasma membrane. The main barrier to diffusion is the perineurium. Further, nerves are composed of both neuronal tissues and non-neuronal tissues, such as connective or fatty tissues, and blood vessels. When performing a popliteal sciatic nerve block for example, it should be kept in mind that here, approximately 60% of the nerve cross-section is non-neuronal tissue.

Structure of the Axonal Membrane
Biologic membranes consist of a molecular lipid bilayer containing proteins adsorbed on the surfaces, as well as embedded in or spanning the hydrocarbon core ( Fig. 29.5 ). The character of the bilayer is determined by the phospholipids, which have long hydrophobic fatty acyl tails that lie in the center of the membrane, as well as by the polar hydrophilic head groups, which are usually composed of zwitterionic portions (containing positive and negative charges) that project into the cytoplasm or the extracellular fluid. Within the membrane there is both lateral and rotational diffusion, which allows lipids and certain proteins to migrate in a fluid mosaic, but most membrane proteins are fixed within specific regions of a membrane, anchored by connections to specific proteins of the cell’s cytoskeleton. A dynamic interaction exists between the cell’s membrane and cytoplasm. Although we focus here on the channel-blocking actions of local anesthetics, it is noteworthy that many other cellular activities, including both metabolic and signal transduction pathways, are modulated by these drugs.

Physiology of Nerve Conduction
The neural membrane is able to maintain a voltage difference of −60 to −90 mV between the intracellular medium and the cell’s outside, because at rest it is relatively impermeable to sodium ions (Na + ) but selectively permeable to potassium ions (K + ). The Na + /K + pump, an active, energy-dependent mechanism, sustains the ion gradients that drive this potential difference by constant extrusion of sodium from within the cell in exchange for a net uptake of potassium, with adenosine triphosphate used as an energy source. Although the membrane is relatively permeable to potassium ions, an intracellular-to-extracellular potassium ratio of 150 to 5 mM, or 30:1, is maintained by active removal of potassium as it leaks passively across the plasma membrane into the cell. The nerve at rest behaves largely as a “potassium electrode,” according to the Nernst equation:
Em≈Ek=(−RTF)ln([K+]i[K+]o)
Ek=−58log30or−85.7mV
An opposite situation exists for Na + , which is at higher concentration outside the cell and has a Nernst potential, E Na , of approximately +60 mV. During an action potential, the nerve membrane transiently switches its higher permeability from K + to Na + , thereby changing the membrane potential from negative to positive, and back again. The progress of this potential change and the underlying events are graphed in Fig. 29.6 . They provide a basis for understanding local anesthetic conduction block.

Permeation of ions through membranes occurs via specialized proteins called ion channels. The conformation of these channels is often sensitive to the membrane potential; both Na + and K + channels in nerve membranes are activated to an “open” conformation by membrane depolarization. Sodium channels, in addition, close to an “inactivated” conformation after their initial activation. A small membrane depolarization extending along an axon from a region of excited membrane, will begin to open both Na + and K + channels. The Na + channels open faster, and the inwardly directed Na + current (see Fig. 29.6 ) depolarizes the membrane further, thereby leading to opening of more Na + channels and increasing the inward Na + current even further ( Fig. 29.7 ). This sequence of events continues during the depolarizing phase until some of the Na + channels have become inactivated and enough of the K + channels have opened to change the balance of current and result in a net outward current that produces membrane repolarization (see Fig. 29.7 ). After one action potential, the concentrations of Na + and K + have changed little for the large myelinated fibers but by as much as 10% for the small, nonmyelinated axons. The Na + ions entering and K + ions leaving the cell as a result of this process are restored by the Na + /K + pump.

Depolarizations too weak to activate enough Na + channels to produce a net inward current are below the membrane’s excitability threshold . The precise value of the threshold varies in different regions of the cell and can change with time. Directly after an impulse, when some Na + channels are still inactivated and some K + channels are still activated, the threshold is above its “resting” value and the membrane is “refractory” to stimulation. Over time, as Na + inactivation decays and K + channels return to their closed conformation, the original resting threshold value is restored. The action potential is a wave of depolarization that is propagated along the axon by continuous coupling between excited and nonexcited regions of membrane. Ionic current (the action current) enters the axon in the excited, depolarized region and then flows down the axoplasm and exits through the surrounding membrane, thereby passively depolarizing this adjacent region (see Fig. 29.3 ).
Although this local circuit current spreads away from the excited zone in both directions, the region behind the impulse, having just been depolarized, is absolutely refractory, and propagation of impulses is thus unidirectional. The local circuit current spreads rapidly along a length of insulated internode in a myelinated axon (see Fig. 29.3 ), and many nodes of Ranvier in sequence are depolarized to threshold with little intervening delay. Single impulses do not jump from node to node as separate, discrete events; instead, active depolarization occurs simultaneously along several centimeters of the largest axons (see Fig. 29.3 ). Indeed, the local circuit current is so robust that it can skip past two completely nonexcitable nodes (e.g., blocked by local anesthetic) and successfully stimulate a third node. If excitability is partially reduced, such as by inhibition of some of the Na + channels, the amplitude of impulses in successive nodes falls accordingly, a process that can continue for many centimeters. This situation probably occurs during certain phases of local anesthesia, as discussed later. When enough of the Na + channels are blocked, local circuit current fails to bring the adjacent resting region to threshold, and the impulse is fully extinguished.
Mechanism of Action of Local Anesthetics (Pharmacodynamics)
Active Form
Local anesthetic bases are poorly to sparingly soluble in water but are quite soluble in relatively hydrophobic organic solvents. Therefore as a matter of chemistry (and to optimize shelf life), most of these drugs are formulated as hydrochloride salts. The p K a of the drug and tissue pH determine the amount of drug that exists in solution as free base or as positively charged cation when injected into living tissue (see earlier). Furthermore, uptake of the drug by tissue, largely via lipophilic adsorption, will also alter its activity, both by shifting the effective p K a downward, thereby favoring the neutral base form, and by limiting diffusion of the anesthetic away from the site of injection. Moderately hydrophobic local anesthetics block faster than either hydrophilic or highly hydrophobic ones, delivered at the same concentration, for the following reasons. Moderately hydrophilic local anesthetic block, such as lidocaine, are less bound to tissues than very hydrophobic drugs are (e.g., tetracaine) but are still more membrane permeant than very hydrophilic ones (e.g., 2-chloroprocaine). The highly hydrophobic local anesthetics, having higher intrinsic potencies (see Table 29.2 ), are therefore used in lower concentrations and their diffusion-controlled rate of onset is correspondingly reduced.
Which form of the local anesthetic, charged cation or neutral base, is actually responsible for blockade of impulses? More alkaline solutions of local anesthetics block nerve conduction more effectively. On sheath-free nerves, the rate of inhibition by tertiary amine anesthetics is greater at alkaline than at neutral external pH because membrane permeation, favored by the base over the cationic species, determines the rate of access to the binding site. Direct control of axoplasmic pH (or internal perfusion with permanently charged quaternary amine homologs) shows that the dominant potency derives from the cationic species acting from the cytoplasmic surface. The uncharged base also has intrinsic pharmacologic activity, however, which explains the effectiveness of benzocaine as a topical local anesthetic. The local anesthetic attaches to the binding site with its aromatic moiety, while the charged portion protrudes into the sodium channel’s lumen.
Electrophysiologic Effect of Local Anesthetics
The resting membrane potential of nerve is little affected by local anesthetics. As the concentration of local anesthetic applied to the nerve is increased, a decrease in the rate of depolarization and in the peak amplitude of the action potential occurs until the impulse is abolished. By using a “voltage-clamp” procedure, Na + currents and their inhibition by local anesthetics can be directly assayed ( Fig. 29.8 A ). When the membrane of isolated neurons is rapidly depolarized to a constant value, the time course of ionic currents is observed. Sodium currents during one initial depolarization are reduced by subclinical doses of local anesthetic (e.g., 0.2 mM lidocaine) and totally abolished by clinical doses (e.g., 1% lidocaine ≈40 mM). If the test depolarization is applied repeatedly at frequencies higher than 5 Hz (five pulses per second), the partially depressed ( tonically inhibited) Na + current is further reduced incrementally for each pulse until a new steady-state level of inhibition is reached. This frequency-dependent inhibition, also called phasic inhibition , is reversed when stimulation is slowed or stopped, and currents return to the level of tonic inhibition observed in the resting nerve. Paralleling the phasic inhibition of Na + currents in voltage-clamped membranes is a “use-dependent” blockade of action potentials during normal physiologic function (see Fig. 29.8 B ).

Phasic actions are a manifestation of the selective affinity of local anesthetics for conformations of the Na + channel that result from depolarization. Both “open” and “inactivated” states of the channel bind local anesthetics more avidly than the resting state does. Repeated depolarization thus increases the fraction of drug-bound channels; dissociation of these bound drug molecules is usually a slower process than the normal recovery from inactivation (see earlier) and results in the use-dependent accumulation of channels in the blocked condition and the phenomenon of phasic block.
By its selective binding to a channel state, the local anesthetic stabilizes that state. During phasic block, therefore, more inactivated channels become drug bound, and reciprocally, less activation can occur. This relationship between state-dependent affinities and modification of transitions among states through drug binding is known as the “modulated receptor” model. Overall binding of anesthetic is increased by membrane depolarization for two reasons: more binding sites become accessible during activation (the “guarded receptor” model) and drug dissociation from inactivated channels is slower than from resting channels (the modulated receptor model).
The potency of local anesthetics to produce tonic and phasic inhibition is similarly dependent on their structure, hydrophobicity, and p K a . There appears to be a single, albeit complex, binding site for local anesthetics on the Na + channel, with a “tonic” affinity at rest and increased “phasic” affinity occurring as a result of depolarization. The sodium channel can be influenced by a number of drugs or toxins/venoms, and the different sites are numbered. The binding site for local anesthetics is referred to as Site 9, while the outer channel pore, binding site for tetrodotoxin (TTX) or saxitoxin (STX), is referred to as Site 1.
The Nature of the Local Anesthetic
Binding Site
Intentional mutation of specific amino acids of the Na + channel has allowed definition of regions that interact directly with local anesthetics. The major functional protein of the Na + channel (the α-subunit) is composed of four homologous “domains” (D-1 to D-4), each of which contains six helical regions (S1 to S6) that span the core of the membrane ( Fig. 29.9 A ). Each domain also has a loop, termed the “P region,” that links the extracellular ends of its S5 and S6 transmembrane segments; the P regions extend inward between the transmembrane regions such that when the α-subunit folds together, each P loop contributes a quarter of the cylindrical ion “selectivity pore,” the narrowest passage of an open channel (see Fig. 29.9 B ). Voltage sensitivity derives from the positive charges located on S4 segments, which slide or swing “outward” in response to membrane depolarization. By linkages still unknown, this movement of S4 results in a conformational rearrangement of the S6 segments, which form the inner, cytoplasmic entry to the channel. Closed-to-open channel gating results from movement of the S6 segments, whereas inactivation gating results from binding of the cytoplasmic loop located between D-3 and D-4 to the cytoplasmic opening of the channel.

Local anesthetics bind in the “inner vestibule” of the closed Na + channel (see Fig. 29.9 C ). Amino acid mutations in the S6 segments of D-1, D-3, and D-4 all modify local anesthetic action, thus suggesting either that these regions form a “pharmacophore” small enough to simultaneously contact the drug at three surfaces or that the local anesthetic molecule moves rapidly among these three segments. The rate constant for binding of local anesthetic to the closed Na + channel is larger for the more hydrophobic molecules, which suggest that drug molecules can reach the binding site (and depart from it) through a “hydrophobic” pathway. The charged species of local anesthetics dissociates much more slowly from closed and inactivated Na + channels than the neutral form does, which suggests that an ionic bond may be involved in drug binding or that the charged molecule moves only slowly along the hydrophobic pathway. In brief, hydrophobicity delivers the drug to the receptor and charge keeps it there.
Neurophysiologic Aspects of Phasic Inhibition
Different fiber types in the nerve are affected differently during local anesthesia. At least part of this difference arises from pharmacokinetic factors. At the onset of and during recovery from clinical block, in particular, longitudinal and radial diffusion of drug will produce concentration variations within and along the nerve. This variation is superimposed on the dynamic use-dependent inhibition to provide variable propagation, which depends on a fiber’s geometry, position within the nerve, and functional as well as electrophysiologic properties.
Different fiber types are also differentially sensitive to local anesthetic blockade. In vivo experiments in which continuous superperfusion of peripheral nerve allows equilibration with drug, and experiments in which a drug bolus is delivered by percutaneous injection, analogous to clinical peripheral nerve block, show that small myelinated axons (Aγ motor and Aδ sensory fibers) are the most susceptible to impulse suppression. Next in order of block are the large myelinated (Aα and Aβ) fibers, and the least susceptible are the small, nonmyelinated C fibers. In fact, in this last group, impulses in the slowest conducting population (conduction velocity of 0.5-0.8 m/s) are the most resistant to local anesthetic. Clinically, testing of block efficacy is often performed using methods that target Aδ sensory fibers, so these findings suggest that loss of temperature or pin-prick discrimination does not guarantee complete and reliable block of all sensory modalities.
Selective Block of Na + Channel Isoforms
Nine different mammalian Na + channels have been physiologically identified and their genes have been sequenced. At least four of them are found in peripheral neurons, and some are exclusively associated with nociceptive afferents. Obviously, it would be clinically advantageous to selectively inhibit these channels and thus prevent or reduce pain while sparing other functions. Although selective channel blockade has been attained with naturally occurring small peptide toxins, relatively little selective blockade by local anesthetics has been reported, probably because the local anesthetic pharmacophore is too similar among the different channel isoforms and the local anesthetic molecules themselves have several rotational axes, which makes them poor structural templates for selecting among static binding pockets. Selectively blocking different sodium channel isoforms can lead to distinct effects because they are not evenly distributed across the nerve and react differently to activation. Specifically, one of the main functions of the Nav1.7 isoform is to act as an amplifier in the terminals of primary sensory neurons, while Nav1.8 is vital to the repetitive firing in these neurons, and Nav1.9 is able to generate persistent currents which can increase membrane excitability. Some recent advances which hold promise to improve nerve block properties of local anesthetics are summarized toward the end of this chapter.
Sodium Channel Isoforms and Their Contribution to Human Diseases Characterized by Pain or Pain Insensitivity
We now know several mutations in the prototypical neuronal sodium channels (Nav1.7, 1.8, and 1.9) which can lead to states of either spontaneous pain or profound and selective impairment of pain sensitivity. This depends on the type of mutation and mode of inheritance. For example, mutations in Nav1.7 may lead to loss of channel function and, in the most extreme form, congenital insensitivity to pain. In contrast, activating mutations in the same channel can trigger erythromelalgia or paroxysmal extreme pain disorder. Molecular studies reveal that these disorders involve several distinct types of mutations in NaV1.7 sodium channels. When these channels are inserted into cells that lack sodium channels, they generate spontaneous, temperature-sensitive inward currents. Another recent finding was that certain preclinical models of visceral pain (e.g., cystitis) are not responsive to therapies specifically targeting the NaV1.7 channels, while block of NaV1.9 channels was effective. Lastly, it is important to note that mutations in nonneuronal sodium channels can have profound clinical consequences as well. For example, mutations of the Nav1.4 sodium channel isoform in skeletal muscle can produce myotonia, periodic paralysis, and congenital myasthenia.
Aberrant impulses, which are often considered the hallmarks of various diseases of excitable membranes, such as abnormal repetitive firing in neuropathic pain or in certain types of inherited skeletal myotonia, are abolished by systemic lidocaine in doses that do not block normal propagating impulses. Conditions for the sensitivity of such impulses to local anesthetics, such as lidocaine, appear to result from the patterns of impulse spikes superimposed on slow membrane depolarizations caused by abnormal expression of Na + channels rather than from selective sensitivity of certain subtypes of channels to these drugs.
Summary of Local Anesthetic Mechanisms
Impulse blockade by local anesthetics may be summarized by the following chronology:
- 1.
Solutions of local anesthetic are deposited near the nerve. Removal of free drug molecules away from this locus is a function of tissue binding, removal by the circulation, and local hydrolysis of aminoester anesthetics. The net result is penetration of the nerve sheath by the remaining free drug molecules.
- 2.
Local anesthetic molecules then permeate the nerve’s axon membranes and reside there and in the axoplasm. The speed and extent of these processes depend on a particular drug’s p K a and on the lipophilicity of its base and cation species.
- 3.
Binding of local anesthetic to sites on voltage-gated Na + channels prevents opening of the channels by inhibiting the conformational changes that underlie channel activation.
- 4.
During onset of and recovery from local anesthesia, impulse blockade is incomplete and partially blocked fibers are further inhibited by repetitive stimulation, which produces an additional, use-dependent binding to Na + channels.
- 5.
One local anesthetic binding site on the Na + channel may be sufficient to account for the drug’s resting (tonic) and use-dependent (phasic) actions.
- 6.
The clinically observed rates of onset and recovery from blockade are governed by the relatively slow diffusion of local anesthetic molecules into and out of the whole nerve, not by their much faster binding and dissociation from ion channels. A clinically effective block that may last for hours can be accomplished with local anesthetic drugs that dissociate from Na + channels in a few seconds.
Clinical Pharmacology
Successful use of regional anesthesia requires knowledge of the pharmacologic properties of the various local anesthetic drugs, as well as technical skill in performance of the nerve block. Local anesthetic requirements vary considerably, depending on factors such as the type of block, surgical procedure, and physiologic status of the patient.
Commonly used aminoester local anesthetics include procaine, chloroprocaine, tetracaine, and the first true local anesthetic, cocaine. Commonly used aminoamides include lidocaine, mepivacaine, prilocaine, bupivacaine (the racemic form and its levoenantiomer), ropivacaine, and etidocaine. The ester and amide local anesthetics differ in their chemical stability, locus of biotransformation, and allergic potential. Amides are extremely stable, whereas esters are relatively unstable in solution. Aminoesters are hydrolyzed in plasma by cholinesterase enzymes, but the amides undergo enzymatic degradation in the liver. Two exceptions to this trend include cocaine, an ester that is metabolized predominantly by hepatic carboxylesterase, and articaine, an amide local anesthetic widely used in dentistry that is inactivated by plasma carboxylesterase-induced cleavage of a methyl ester on the aromatic ring.
p -Aminobenzoic acid is one of the metabolites of ester-type compounds that can induce allergic-type reactions in a small percentage of patients. The aminoamides are not metabolized to p -aminobenzoic acid, and reports of allergic reactions to these agents are extremely rare.
General Considerations
Clinically important properties of the various local anesthetics include potency, speed of onset, duration of anesthetic action, and differential sensory/motor blockade. As previously indicated, the profile of individual drugs is determined by their physicochemical characteristics (see Table 29.2 ).
Anesthetic Potency
Hydrophobicity appears to be a primary determinant of intrinsic anesthetic potency because the anesthetic molecule must penetrate into the nerve membrane and bind at a partially hydrophobic site on the Na + channel. Clinically, however, the correlation between hydrophobicity and anesthetic potency is not as precise as in an isolated nerve. Differences between in vitro and in vivo potency may be related to a number of factors, including local anesthetic charge and hydrophobicity (which influence partitioning into and transverse diffusion across biologic membranes) and vasodilator or vasoconstrictor properties (which influence the initial rate of vascular uptake from injection sites into the central circulation).
Onset of Action
The onset of conduction block in isolated nerves is related to the physicochemical properties of the individual agents. In vivo latency is also dependent on the dose or concentration of local anesthetic used. For example, 0.75% bupivacaine has more rapid onset than 0.25%. Chloroprocaine demonstrates a rapid onset of action in humans because its low systemic toxicity permits its use in high concentrations (3%).
Duration of Action
The duration of action of the various local anesthetics differs markedly. Procaine and chloroprocaine have a short duration of action. Lidocaine, mepivacaine, and prilocaine produce a moderate duration of anesthesia, whereas tetracaine, bupivacaine, ropivacaine, and etidocaine have the longest durations.
In humans, the duration of anesthesia is markedly influenced by the peripheral vascular effects of the local anesthetic drugs. Many local anesthetics have a biphasic effect on vascular smooth muscle; at low concentrations these agents tend to cause vasoconstriction, whereas at higher, clinically administered concentrations, they cause vasodilation. However, differences exist in the degree of vasodilator activity of the various drugs. The effects of local anesthetics on vascular tone and regional blood flow are complex and vary according to concentration, time, and the particular vascular bed near the site of application. As a practical example, the topical local anesthetic formulation EMLA (eutectic mixture of the local anesthetics lidocaine and prilocaine) vasoconstricts cutaneous vessels initially and through most of the first hour of application, but vasodilation is observed after 2 or more hours of application.
Differential Sensory and Motor Blockade
Another important clinical consideration is the ability of local anesthetic agents to cause differential inhibition of sensory and motor activity. Bupivacaine became popular in the 1980s for epidural blocks because it was better than the previously available long-acting agents (e.g., etidocaine) in producing adequate antinociception without profound inhibition of motor activity, particularly when dilute solutions are used. Bupivacaine is widely used epidurally for obstetric analgesia and postoperative pain management because it can provide acceptable analgesia with only mild muscle weakness. Additional observations on the sensory selectivity of newer local anesthetics are detailed later in the section on chiral local anesthetics.
Traditional texts often state that small-diameter axons, such as C fibers, are more susceptible to local anesthetic block than larger-diameter fibers. However, when careful measurements are made of single-impulse annihilation in individual nerve fibers, exactly the opposite differential susceptibility is noted (see earlier). Repetitive stimulation, such as occurs during propagation of trains of impulses, produces further, phasic inhibition of excitability, but it is not clear how this will affect a functionally selective failure of impulses. The length of drug-exposed nerve in the intrathecal space, imposed by anatomic restrictions, can perhaps explain clinically documented differential spinal or epidural blockade, with longer drug-exposed regions yielding block by lower concentrations of local anesthetic. However, this reasoning does not explain the functionally differential loss from peripheral nerve block. Other factors may include actual spread of the drug along the nerve or its selective ability to inhibit Na + channels over K + channels, which in itself can produce a differential block because these channels are present in very different proportions in different types of nerves. As a result of these confounding factors, conclusions about fiber-type involvement in chronic pain syndromes based on the dose or concentration requirement for pain relief in diagnostic nerve blockade should not be made.
Factors Influencing Anesthetic Activity in Humans
Dosage of Local Anesthetic
As the dosage of local anesthetic is increased, the probability and duration of satisfactory anesthesia increases and the time to onset of block is shortened. The dosage of local anesthetic can be increased by administering either a larger volume or a more concentrated solution. For example, increasing the concentration of epidurally administered bupivacaine from 0.125% to 0.5% while maintaining the same volume of injectate (10 mL) resulted in shorter latency, an improved incidence of satisfactory analgesia, and a longer duration of sensory analgesia. The volume per se of anesthetic solution probably influences the spread of anesthesia. For example, 30 mL of 1% lidocaine administered into the epidural space produced a level of anesthesia that was 4.3 dermatomes higher than that achieved when 10 mL of 3% lidocaine was given.
In selecting the volume and concentration for a specific block in a particular patient, clinicians must balance the risk of adverse effects from excessive dosing (e.g., systemic toxicity, excessive motor or autonomic blockade) against the increased risk of block failure if an inadequate volume or concentration is chosen. The degree to which additional volume can compensate for imprecise needle placement varies among different blocks. With the advent of very precise needle placement using ultrasound-guided blockade, it has become clear that the median effective volume for obtaining successful blockade can be achieved with smaller volumes than have been recommended from some previous clinical series based on traditional methods of needle localization. For example, in a recent dose-finding randomized trial for femoral nerve blockade, the median volumes for 50% or 95% success in obtaining dense sensory and motor block at 30 minutes under ultrasound guidance were 57% and 54% of the corresponding volumes with the use of nerve stimulation. Interested readers will note the very wide confidence intervals found in this study and are encouraged to read recent work on some statistical design issues for dose-response studies involving all types of anesthetics. As limited by toxicity considerations, the aim in most clinical situations should be to choose doses that provide high success rates; that is, an effective dose (ED) in 95% of patients (ED 95 ) is generally a more relevant guide to dose selection than an ED 50 dose. These considerations are especially salient when performing regional anesthesia for patients with chronic pain, hyperalgesia, or a history of previous failed regional anesthesia. Also, decreasing the dose substantially may provide a satisfactory block after 30 minutes (a common endpoint for clinical studies), but at the same time, the block may have a decreased duration of action. Duration of action is important such that the block will cover the intensely painful period after surgery, and if this period is longer than could be achieved using plain local anesthetics, and in cases where continuous techniques are not feasible, adjuvants/additives may help in prolonging the block.
Addition of Additives
Epinephrine
Vasoconstrictors, usually epinephrine, are frequently included in local anesthetic solutions to decrease the rate of vascular absorption, thereby allowing more anesthetic molecules to reach the nerve membrane and thus improve the depth and duration of anesthesia. The use of epinephrine as a marker for inadvertent intravascular injection continues to be sensible, even though false negatives and false positives can occur, such as difficulty in interpretation for specific patient groups as with parturients and patients under anesthesia or on β-blockers. Clinically used solutions typically contain 5 μg/mL or 1:200,000 of epinephrine, reflecting a balance between efficacy and vasoconstriction versus systemic side effects of epinephrine. The extent to which epinephrine prolongs the duration of anesthesia depends on the specific local anesthetic used and the site of injection. Epinephrine will significantly extend the duration of both infiltration anesthesia and peripheral nerve blockade with shorter-duration agents (e.g., lidocaine); epinephrine produces mild intensification of blockade but only most modest prolongation of epidural or peripheral blocks with bupivacaine. Activation of α 2 -adrenergic receptors in the spinal cord may contribute to the beneficial effect of epidural epinephrine.
Clonidine and Dexmedetomidine
The α-2 agonist clonidine prolongs the action of local anesthetics by about 2 hours with wide variation between studies , and its conjectured mechanisms of action include actions on α-2 receptors and on hyperpolarization-induced currents. However, there is a large number of negative studies, and adverse systemic events are of concern, including hypotension, bradycardia, and sedation, such that limiting the clonidine dose to 0.5 to 1 μg/kg of ideal body weight has been proposed. Dexmedetomidine is a much more specific α-2 agonist, and prolongs both motor and sensory block by long-acting local anesthetics by approximately 4 hours. Similar to clonidine, dexmedetomidine has also been shown to block the hyperpolarization-induced current. Nevertheless, risk of systemic adverse effects remains high, and optimal doses have not been determined. There seems to be no increased risk of neurotoxicity when clonidine or dexmedetomidine are used as nerve block adjuvants.
Buprenorphine
The partial μ-opiate receptor agonist, buprenorphine, intensifies blockade by two mechanisms, namely blockade of κ- and δ-opioid receptors, and blockade of voltage-gated sodium channel-blocking properties. Blockade by long-acting local anesthetics is prolonged by about 6 hours, but at the price of a high incidence of nausea and vomiting, such that the use of buprenorphine has largely been abandoned. Buprenorphine is considered safe in terms of neurotoxicity.
Dexamethasone
The most effective adjuvant for prolonging block duration with minimal side effects currently available is dexamethasone, able to prolong duration of medium-acting local anesthetics by 2 to 3 hours, and the block of long-acting local anesthetics by up to 10 hours on average. Blocks can be prolonged by intravenous or perineural administration of dexamethasone. Despite the fact that perineural administration of dexamethasone seems to be more effective than systemic use, many providers use systemic dexamethasone to avoid mixing drugs which were not designed to be administered together, circumvent the problem of off-label perineural use, and profit from the antiemetic effects of systemic dexamethasone. Doses between 4 and 10 mg have typically been used in adults. The precise mechanism of action of dexamethasone is not understood and the potential for neurotoxic side effects has not been adequately studied.
Site of Injection
The most rapid onset but the shortest duration of action occurs after intrathecal or subcutaneous administration of local anesthetics. The longest latencies and durations are observed after conventional non–ultrasound-guided large-volume brachial plexus blocks. For example, intrathecal bupivacaine will usually produce anesthesia within 5 minutes that will persist for 3 to 4 hours. However, when bupivacaine is administered for brachial plexus blockade, the onset time is approximately 20 to 30 minutes, and the duration of anesthesia (or at least analgesia) averages 10 hours. These differences in the onset and duration of anesthesia and analgesia are due in part to the particular anatomy of the area of injection, which will influence the rate of diffusion and vascular absorption and, in turn, affect the amount of drug used for various types of regional anesthesia. In the subarachnoid space, for example, the lack of a nerve sheath around the spinal cord and deposition of the local anesthetic solution in the immediate vicinity of the spinal cord are responsible for the rapid onset of action, whereas the relatively small amount of drug used for spinal anesthesia probably accounts for the short duration of conduction block.
In contrast, the onset of brachial plexus blockade is slow because the anesthetic agent is usually deposited at some distance from the nerve and must diffuse through various tissue barriers before reaching the nerve membrane. The prolonged block with brachial plexus blockade may be related to several factors, including comparatively slow rates of vascular absorption from the brachial plexus sheath, larger doses of drug required for this regional anesthetic technique, and comparatively long segments of nerves exposed to local anesthetic.
Carbonation and pH Adjustment of Local Anesthetics
The addition of sodium bicarbonate to a solution of local anesthetic applied to an isolated nerve accelerates the onset and decreases the minimum concentration ( C m ) required for conduction blockade by increasing pH and increasing the share of uncharged local anesthetic molecules that can more easily diffuse into the nerve cell. Although the effect of carbon dioxide on local anesthetic activity is easily demonstrable in isolated nerve, controversy exists concerning the clinical utility of carbonated local anesthetic solutions and the widespread introduction of ultrasound-guided nerve blockade with faster and more reliable block onset has decreased the relevance of carbonation, at least for peripheral nerve blockade.
Mixtures of Local Anesthetics
Mixtures of local anesthetics for regional anesthesia are sometimes used in an effort to compensate for the short duration of action of certain rapidly acting agents such as chloroprocaine and lidocaine, and the long latency of longer-acting agents such as tetracaine and bupivacaine. Mixtures of chloroprocaine and bupivacaine theoretically offer significant clinical advantages because of the rapid onset and low systemic toxicity of chloroprocaine and the long duration of action of bupivacaine; however, clinical results in studies of combinations have been mixed. The use of catheter techniques for many forms of regional anesthesia makes it possible to begin with a rapid-onset local anesthetic such as lidocaine, mepivacaine, or chloroprocaine and then follow with an infusion of either a shorter-acting or longer-acting local anesthetic thereafter. Clinicians should be cautioned to not use maximum doses of two local anesthetics in combination in the mistaken belief that their toxicities are independent. Toxicity in fact, is additive. Moreover, the use of ultrasound-guided nerve blockade has, in general, led to a decreased onset time and has made mixing local anesthetics less clinically relevant.
Pregnancy
The spread and depth of epidural and spinal anesthesia are greater in pregnant than in nonpregnant women. The effects of pregnancy on local anesthetic potency may reflect a combined effect of mechanical factors associated with pregnancy (i.e., dilated epidural veins decrease the volume of the epidural and subarachnoid spaces) and direct effects of hormones, especially progesterone, on the susceptibility of nerves to conduction blockade by local anesthetics. Hormonal alterations are probably the more important of these two factors because greater spread of epidural anesthesia is already observed during the first trimester of pregnancy, before any gross change in vascular dimensions within the epidural or subarachnoid spaces. The dosage of local anesthetics should probably be reduced in patients in all stages of pregnancy.
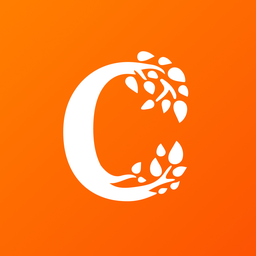
Full access? Get Clinical Tree
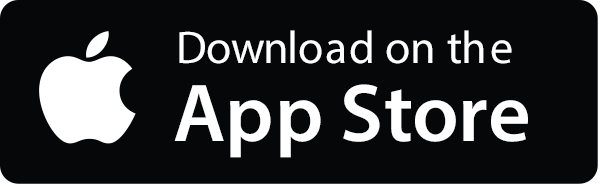
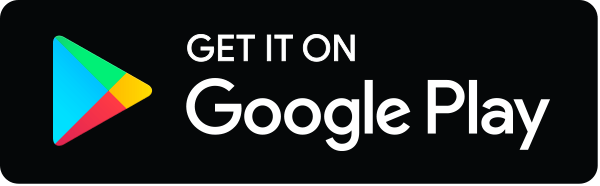