Abstract
One of the main functions of the liver is to protect the organism against intoxication. The endoplasmic reticulum of liver cells contains enzymes that protect the organism against an accumulation of lipid-soluble exogenous and endogenous compounds. This is done by transforming the compounds to water-soluble metabolites, which are then easily excreted by the kidney. Gastrointestinal absorption of orally administered drugs and pharmacology of the gastrointestinal tract is an equally complex system mediated by neuronal and hormonal influences.
Keywords
liver, gastrointestinal, pharmacology, first pass, hepatic extraction, bioavailability
Chapter Outline
Anesthetic Drugs and the Liver
Anesthetic Agents and Hepatic Blood Flow
Metabolism of Volatile Anesthetics and Hepatotoxicity
Metabolism of Intravenous Anesthetic Agents
Hepatobiliary Metabolism and Elimination of Neuromuscular Blockers
Gastric Acid–Suppressing Medications
Drugs to Reduce Portal Venous Pressure
Opioids and the Gastrointestinal System
Other Treatments for Dyslipidemia
Suppression of Gastric Acid Secretion
Direct-Acting Antivirals for Hepatitis C Treatment
PCSK9 Inhibitors for Dyslipidemia
One of the main functions of the liver is to protect against toxins. The endoplasmic reticulum of hepatocytes contains families of enzymes that protect the organism against an accumulation of lipid-soluble exogenous and endogenous compounds. This is done by transforming compounds to water-soluble metabolites, which are more readily excreted by the kidneys. Gastrointestinal absorption of orally administered drugs and the pharmacology of the gastrointestinal tract are critical to pharmacotherapy and to perioperative medicine.
Liver Pharmacology
Cytochrome P450 Enzymes
The bulk of liver drug metabolism is carried out by the cytochrome P450 (CYP 450) enzyme system ( Tables 32.1 and 32.2 ) (see Chapter 4 ). CYP 450 enzymes in the endoplasmic reticulum oxidate lipid-soluble compounds in phase I and phase II reactions. Drug response varies from 25% to 60% among patients because of environmental, genetic, and disease influences. Phase I reactions transform lipophilic molecules into hydrophilic molecules. Phase II reactions then conjugate drugs and metabolites to highly polar compounds that are more readily excreted.
CYP Enzyme | Tissue |
---|---|
1A1 a | Lung, kidney, gastrointestinal tract, skin, placenta, lymphocytes, and others |
1B1 | Skin, kidney, mammary, prostate, uterus, fetus |
2A6 | Lung, nasal membrane, and possibly others |
2B6 | Gastrointestinal tract, lung |
2C | Gastrointestinal tract (small intestine mucosa), larynx, lung |
2D6 | Gastrointestinal tract |
2E1 | Lung, placenta, and others |
2F1 | Lung, placenta |
2J2 | Heart |
3A | Gastrointestinal tract, placenta, fetus, uterus, kidney, lung |
4B1 | Lung, placenta |
4A11 | Kidney |
CYP Enzyme | Sensitive Substrates | Moderately Sensitive Substrates | Strong Inhibitors | Moderate Inhibitors | Strong Inducers | Moderate Inducers |
---|---|---|---|---|---|---|
1A2 | Alosetron, caffeine, duloxetine, melatonin, ramelteon, tasimelteon, theophylline, tizanidine | Clozapine, pirfenidone, ramosetron | Ciprofloxacin, enoxacin, fluvoxamine, zafirlukast | Methoxsalen, mexiletine, oral contraceptives | — | Phenytoin, rifampin, ritonavir, smoking, teriflunomide |
2B6 | Bupropion | Efavirenz | — | — | Carbamazepine | Efavirenz, rifampin, ritonavir |
2C8 | Repaglinide | Montelukast, pioglitazone, rosiglitazone | Clopidogrel, gemfibrozil | Deferasirox, teriflunomide | — | Rifampin |
2C9 | Celecoxib | Glimepiride, phenytoin, tolbutamide, warfarin | — | Amiodarone, felbamate, fluconazole, miconazole, piperine | — | Aprepitant, carbamazepine, enzalutamide, rifampin, ritonavir |
2C19 | S-mephenytoin, omeprazole | Diazepam, lansoprazole, rabeprazole, voriconazole | Fluconazole, fluoxetine, fluvoxamine, ticlopidine | — | Rifampin, ritonavir | Efavirenz, enzalutamide, phenytoin |
2D6 | Atomoxetine, desipramine, dextromethorphan, nebivolol, nortriptyline, perphenazine, tolterodine, venlafaxine | Amitriptyline, encainide, imipramine, metoprolol, propafenone, propranolol, tramadol, trimipramine | Bupropion, fluoxetine, paroxetine, quinidine, terbinafine | Cimetidine, cinacalcet, duloxetine, fluvoxamine, mirabegron |
The CYP1, CYP2 and CYP3 families perform almost 80% of oxidative drug metabolism and 50% of drug elimination. Although the liver is the major site of CYP 450–mediated metabolism, small intestine enterocytes are a secondary site. More than 65 commonly used drugs are metabolized by CYP 2D6. Genetic variability in CYP 2D6 metabolism can thereby lead to subtherapeutic or supratherapeutic drug levels and effects. This variability is usually unanticipated because CYP 450 genotyping is not widely available. Slow metabolism by CYP 2D6 is a major factor in warfarin toxicity, for example. The CYP 3A family also metabolizes a wide variety of drugs. Drug-drug interactions expand variability up to 400-fold. CYP 5 catalyzes the isomerization of prostaglandin endoperoxide to thromboxane A, a reaction that leads to platelet aggregation and potentially thrombosis.
Hepatic Extraction
The fraction of drug presented to the liver that is eliminated during a single pass is called the hepatic extraction ratio. The hepatic extraction ratio becomes equal to 1 (unity) when the drug that reaches the liver is completely eliminated. When no drug is eliminated by the liver, the extraction ratio is zero. For oral drugs that are completely absorbed into the portal circulation, bioavailability depends on the extraction ratio. Bioavailability for high–extraction ratio drugs is much less than for low–extraction ratio drugs ( Table 32.3 ).
Low Ratio (<0.3) | Intermediate Ratio (0.3–0.7) | High Ratio (>0.7) |
---|---|---|
Carbamazepine | Aspirin | Alprenolol |
Diazepam | Quinidine | Cocaine |
Indomethacin | Codeine | Desipramine |
Naproxen | Nifedipine | Lidocaine |
Nitrazepam | Nortriptyline | Meperidine |
Phenobarbital | Morphine | |
Phenytoin | Nicotine | |
Procainamide | Nitroglycerin | |
Salicylic acid | Pentazocine | |
Theophylline | Propoxyphene | |
Valproic acid | Propranolol | |
Warfarin | Verapamil |
Hepatic clearance reflects the removal of the drug as it passes through the liver and is the product of hepatic blood flow multiplied by the extraction ratio ( Fig. 32.1 ). If the liver is very efficient in removing a drug (extraction ratio ~1) but blood flow is low, clearance will also be low. At the same time, if the liver is extremely inefficient in removing a drug, clearance will be low even if blood flow is high.

Plasma Protein Binding
Only unbound drug is able to cross membranes and be eliminated. In patients with low plasma proteins, such as those with end-stage liver disease, the proportion of unbound drug increases. If a drug has a low extraction ratio, an increase in the fraction of unbound drug will proportionally increase clearance. Therefore the unbound drug concentration remains constant and no dose adjustment is required. On the other hand, if a drug has a high extraction ratio, as the fraction of unbound drug increases the clearance remains constant. As the unbound concentration increases, toxicity can ensue.
Anesthetic Drugs and the Liver
Anesthetic Agents and Hepatic Blood Flow
Maintaining hepatic perfusion is important, particularly in patients with compromised hepatic function. The hepatic arterial buffer response, which increases hepatic artery flow when portal vein flow is reduced, is impaired with inhaled anesthetics. Table 32.4 summarizes the effects of various anesthetic agents on hepatic blood flow. Halothane and nitrous oxide decrease hepatic blood flow in a dose-dependent fashion. Hepatic blood flow is maintained at 1 minimum alveolar concentration (MAC) of isoflurane and sevoflurane. Whereas desflurane decreases hepatic blood flow in animals, a single-center human study showed increased hepatic blood flow at 1 MAC desflurane compared with isoflurane.
Halothane | Isoflurane | Sevoflurane | Desflurane | Nitrous Oxide |
---|---|---|---|---|
Decreased | Maintained | Maintained | Decreased in animals, possibly increased in humans | Decreased |
Intravenous (IV) and neuraxial anesthetic agents also affect hepatic blood flow. Propofol and opioids increase hepatic blood flow ( Table 32.5 ). Ketamine does not affect hepatic flow, but oxygen delivery is reduced, possibly owing to increased oxygen consumption by other organs. Etomidate, barbiturates, dexmedetomidine, and benzodiazepines decrease hepatic blood flow. Thoracic epidural anesthesia decreases hepatic blood flow, consistent with previous studies of lumbar epidurals.
Propofol | Ketamine | Etomidate | Barbiturates | Dexmedetomidine | Benzodiazepines | Opioids |
---|---|---|---|---|---|---|
Increased | Maintained | Decreased | Decreased | Decreased | Decreased | Increased |
Metabolism of Volatile Anesthetics and Hepatotoxicity
Many volatile anesthetics are oxidized to variable extents by CPY 2E1 in the liver (see Chapter 3 ). Halothane use has fallen out of favor because of concerns about hepatotoxicity, which involves two different mechanisms. The first reaction is a transient benign postoperative transaminase elevation. The second reaction, halothane hepatitis, is a rare autoimmune reaction leading to centrilobular necrosis and acute liver failure with a high mortality rate. Halothane is oxidized, leading to trifluoroacetyl adduct formation, which leads to antibody production in susceptible patients. Repeat exposure to halothane leads to hepatocyte necrosis. Desflurane, isoflurane, and enflurane also undergo oxidation to trifluoroacetyl, with rare reports of autoimmune liver failure. Sevoflurane does not form reactive trifluoroacetyl intermediates and therefore presents a lower concern for immune-mediated hepatitis.
Metabolism of Intravenous Anesthetic Agents
The majority of IV hypnotic agents undergo hepatic metabolism (see Chapter 2 ). Their pharmacokinetics depend on hepatic blood flow, degree of hepatic extraction, and plasma protein binding. Patients with hepatic dysfunction have greater susceptibility to pharmacologic effects owing to decreased hepatic metabolism, decreased hepatobiliary clearance, and low serum protein and albumin concentrations. The liver oxidizes propofol with a very high extraction ratio; therefore elimination depends largely on hepatic blood flow.
Barbiturates are potent CYP 450 inducers. Patients with deficiencies in heme synthesis pathways receiving barbiturates are at risk of inducing aminolevulinic acid synthase, precipitating an attack of acute intermittent porphyria. The liver is centrally involved in the pathogenesis of this disorder, as aminolevulinic acid synthase induction leads to increased demand for hepatic heme and consumption of hepatic CYP 450 enzymes. Furthermore, liver transplantation has been used for treatment of refractory acute porphyria.
The liver oxidizes midazolam to 1-hydroxymethylmidazolam, an active central nervous system depressant metabolite that can accumulate in patients with hepatic or renal dysfunction. By contrast, lorazepam undergoes glucuronidation to inactive metabolites.
Most opioids are metabolized in the liver. Patients with cirrhosis have decreased clearance of opioids, leading to increased accumulation, which can precipitate hepatic encephalopathy. Lower opioid doses and longer intervals between doses are advocated in these patients.
Hepatobiliary Metabolism and Elimination of Neuromuscular Blockers
The hepatobiliary system metabolizes selected neuromuscular blockers. For aminosteroid nondepolarizing agents (rocuronium, vecuronium and pancuronium), prolonged blockade becomes apparent after redosing or continuous infusion, because redistribution is responsible for recovery from a single bolus dose. A higher initial dose of a nondepolarizing neuromuscular blocker may be needed in patients with cirrhosis owing to an increased volume of distribution. Cisatracurium and atracurium undergo chemical degradation independent of the liver and are useful in redosing when rapid recovery is desired. Succinylcholine is metabolized by plasma cholinesterase, a product of the liver; patients with liver disease can have prolonged blockade. Current reversal agents, including suggamadex, do not undergo hepatic metabolism or excretion.
Gastrointestinal Pharmacology
Basic Principles
Gastrointestinal function is controlled by a variety of influences, including extrinsic autonomic innervation, a complicated intrinsic neural system, and multiple hormones such as gastrin, glucagon, and somatostatin. Gastrointestinal motility is a function of nerve inputs, and slow electrical waves generated in the longitudinal layer located in the smooth muscle are required for peristalsis. Drugs targeting the gastrointestinal system tend to focus on decreasing gastric acid secretion, treating nausea and vomiting, and diminishing side effects of slowed motility causing constipation.
Because of convenience, oral drug administration is the preferred route of delivery for many medications, particularly in the outpatient setting. After oral administration of a drug, CYP 450 enzymes can reduce the portion of the dose that reaches the systemic circulation, influencing the drug’s effects, which is known as first-pass metabolism . Oral administration is highly dependent on drug bioavailability, which is determined by the quantity of drug absorbed from the gastrointestinal tract and hindered by first-pass elimination in the gastrointestinal wall, liver, and lung. Gastrointestinal absorption depends on the stability of the drug in gastrointestinal fluids, absorption time, solubility, and how readily the drug permeates the gastrointestinal epithelium ( Fig. 32.2 ). The primary site of drug absorption is the small intestine, followed by the stomach and colon. For about 40% of commonly used drugs, less than half of the administered oral dose is bioavailable because of limited absorption, first-pass metabolism, or both. Oral bioavailability can be altered by drug interactions that result in either inhibition or induction of the involved enzymes. Drug metabolism differences in the intestine and liver between patients are common and are major contributors to differences in drug response, including adverse effects.

When an orally administered drug undergoes extensive first-pass metabolism, its bioavailability in the setting of CYP 3A inhibition can increase severalfold, whereas the rate of elimination can be reduced ( Table 32.6 ). When a drug is administered orally, intestinal CYP 3A enzymes are exposed to higher concentrations of the drug and are inhibited to a greater degree than are unaffected hepatic CYP 3A enzymes. An example of this is an interaction between grapefruit juice and CYP 3A substrates. Grapefruit juice is contraindicated in patients receiving drugs that are extensively metabolized by CYP 3A, including calcium channel blockers and statins, as a single glass can cause CYP 3A inhibition for 24 to 48 hours.
CYP 3A Substrates | CYP 3A Inhibitors | CYP 3A Inducers |
---|---|---|
Calcium Channel Blockers Diltiazem Felodipine Nifedipine Verapamil | Calcium Channel Blockers Diltiazem Verapamil | Rifamycins Rifabutin Rifampin Rifapentine |
Immunosuppressant Agents Cyclosporine Tacrolimus | Azole Antifungal Agents Itraconazole Ketoconazole | Anticonvulsant Agents Carbamazepine Phenobarbital Phenytoin |
Benzodiazepines Alprazolam Midazolam Triazolam | Macrolide Antibiotics Clarithromycin Erythromycin (Not azithromycin) | Anti-HIV Agents Efavirenz Nevirapine |
Statins Atorvastatin Lovastatin (Not pravastatin) | Anti-HIV Agents Delavirdine Indinavir Ritonavir Saquinavir | Others St. John’s wort |
Macrolide Antibiotics | ||
Clarithromycin Erythromycin | ||
Anti-HIV Agents Indinavir Nelfinavir Ritonavir Saquinavir | ||
Others Losartan Sildenafil |
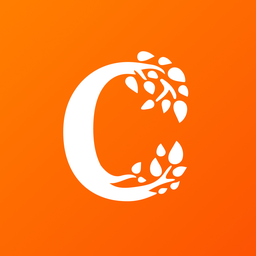
Full access? Get Clinical Tree
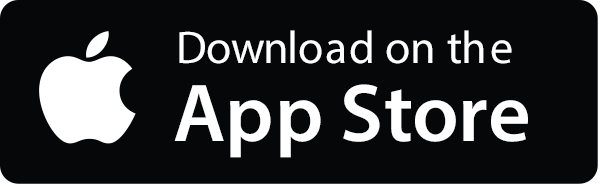
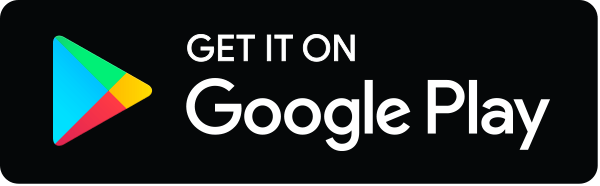
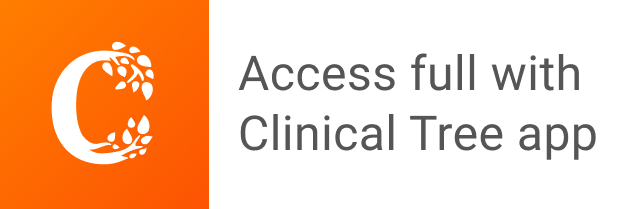