Fig. 5.1
The concept of experimental pain. The pain system can be considered as a “black box” between the experimental stimulation (input) and the response (output). When input and output are reproducible, it is possible to reveal differences in pain processing between, e.g., healthy volunteers and patients. Furthermore, modulation of the pain system is possible through various mechanisms (e.g., medication, modulation, or sensitization) and may provide additional information
Experimental Visceral Pain Stimuli
The natural origin of visceral pain is not fully understood, although a variety of innate stimuli are clearly associated with pain from the viscera. Naturally occurring visceral stimuli are distention of hollow organs, ischemia, inflammation, spasms, and traction of the gut. Also, thermal stimuli (heat and cold) may provoke pain from the viscera although (apart from the esophagus) this seems not to occur under normal physiological conditions [4].
The ideal experimental stimulus to elicit gut pain in humans should mimic innate visceral stimuli, be minimally invasive, reliable in test–retest experiments, and quantifiable. The response to the stimulus should increase with increasing stimulus intensity and preferably the pain should reflect observations in diseased organs by evoking phenomena such as allodynia and hyperalgesia [5, 6]. The different methods currently available for visceral sensory stimulation are:
Electrical stimuli
Mechanical stimuli
Thermal stimuli
Chemical stimuli and models evoking visceral hyperalgesia
Ischemic stimuli are difficult to quantify in human and is normally not used as a direct stimulus. In the following sections, the individual pain stimuli most widely used for visceral QST and experimental-evoked hyperalgesia are briefly discussed.
Electrical Stimulation
Depolarization of visceral nerve afferents by electrical current has been widely used as an experimental stimulus of the human gut. The electrical stimuli have proved to be safe in all parts of the gastrointestinal (GI) tract and are easily controlled over time. As the stimulus by-pass peripheral receptors in the gut wall, the method is used to characterize afferent transmission and central processing of visceral stimuli [1–3, 7]. A major challenge of visceral electrical stimulation is varying electrode contact with gut mucosa. Integration of the electrical stimulation device and a biopsy forceps of an endoscope provide an elegant solution to this problem and allows application of stimuli in well-defined areas throughout the GI tract with high spatial precision.
Mechanical Stimulation
The mechanical properties of the gut are important for its function as a digestive organ and it contains numerous mechanoreceptors distributed mainly in the muscle layers of the gut [8]. Mechanical stimulation of the gut is typically done by distension of a balloon positioned in the segment under investigation. Widely used methods are computerized systems such as the “Barostat,” where balloon pressure and volume can be strictly controlled during distension and thereby transmit a controlled mechanical stimulus [9]. The major advantage of the Barostat system and similar pressure–volume-based methods are the relatively low costs and reliability, making it useful for routine purposes. However, the accuracy of these systems has been questioned mainly due to uncontrolled elongation of the balloon during distension in nonspheric organs such as the rectum. Accordingly, elongation and deformation of the balloon during distention may not reliably reflect mechanoreceptor activation. These problems may be overcome by calculation of the balloon radius and tissue strain using impedance planimetry or imaging-based methods such as ultrasound or magnetic resonance. In accordance with recent studies, strain of the gut is probably the most consistently mechanical parameter relating to mechanoreceptors activation and possibly the subjective sensory response [10]. However, the technical complexity of such systems has so far limited their use to advanced experimental GI research and they are not widely used in the clinical setting.
Thermal Stimulation
Short-lasting thermal stimuli of the human GI tract are believed to activate unmyelinated afferents in the mucosa through the transient receptor potential cation channel subfamily V member 1 (TRPV1) receptor. This is opposed to mechanical and electrical stimuli activating afferents in both superficial and deeper layers in the viscera [8]. Although thermal stimuli of the gut have been used to some extent in animal studies, temperature stimuli in the human GI tract have only been used in a limited number of studies. This has mainly been due to difficulties in controlling the temperature rate (being essential for control of nociceptor activation). However, new technologies for thermal stimulation of the GI tract have been developed. These are based on continuously recirculating of water inside a balloon with concomitant measurement of balloon temperature [11]. The model has been used in many studies unraveling pain mechanisms in patients and was recently modified for use in the lower gut (rectosigmoid) [7]. Based on this method, the temperature stimuli show a linear stimulus–response relationship, thus demonstrating validity of the model. However, uncertainty in pain assessments due to fast increase in temperature (2 °C/min) has been demonstrated and recently it was proposed that individual differences in reaction time could affect the accuracy of rating. Consequently, in future studies a slower temperature increase (0.2 °C/min) is recommended [12, 13].
Chemical Stimulation and Models Evoking Visceral Allodynia and Hyperalgesia
Inflammation of the gut generally leads to altered sensations including pain. This has been investigated experimentally in patients with, e.g., esophagitis [4]. Chemical stimulation of the GI tract more closely resembles clinical diseases and is believed to approach the ideal experimental visceral pain stimulus [14]. Most chemical stimuli are assumed to activate unmyelinated C-fibers. Following chemical stimulation, tissue injury generates release of multiple molecules acting synergistically to produce inflammatory responses and hyperalgesia. Acid stimulation is the most common used method to evoke such visceral hyperalgesia, although chemical stimulation with alcohol, bradykinin, glycerol, capsaicin, and hypertonic saline has also been used to induce gut sensitization [1–3]. To mimic the clinical situation experimentally, it may be necessary to use a mixture of chemical substances with diverse tissue effects. An example of such mixed chemical stimulation is seen in the combination of acid and capsaicin working through different cellular interaction sites. Accordingly, acid targets the TRPV1 receptor extracellularly, whereas the capsaicin targets the TRPV1 receptor predominantly intracellularly. The method has been applied in, e.g., the esophagus of healthy volunteers and provides a human model to study visceral hyperalgesia [12, 13]. Most studies on visceral hyperalgesia have demonstrated increased pain to one or more stimulation modalities after experimentally induced sensitization by chemicals. Also, the duration and magnitude of hypersensitivity has been shown to be related to exposure area and dose of the chemicals [15]. Although chemical stimulation and experimental induced hyperalgesia generally posses high reproducibility in test–retest experiments, it has been demonstrated that the hyperalgesic response to acid is variable comparing the first time a subject is exposed to esophageal acid perfusion with the second time [12, 13]. Therefore, it is recommended to have a training session, where the subjects are introduced and exposed to chemical perfusion in order to familiarize them with the stimulus.
Multimodal Visceral Stimulation
The major limitation of the existing human models for visceral pain stimulation is that they may not mimic clinical pain because they are based on single, short-lasting stimuli only partly involving the many mechanisms typically activated during diseases. Therefore, the basic neurobiological mechanisms in clinical pain may be different from those relating to an experimental stimulation and experimental visceral models mimicking more closely the clinical situation are needed. Such a model should be based on multimodal testing regimens in which different receptors and central nervous system mechanisms are activated. Hence, a test battery where different stimuli are used will increase the probability for activation of a range of relevant nervous mechanisms. Especially if the stimulation is relatively long lasting and includes modalities known to evoke peripheral as well as central sensitization of the nervous system, the likelihood that part of the model will mimic clinical pain is high despite the nonharmful nature of the stimulation. To fulfill these requirements, a multimodal testing approach has been developed for experimental stimulation of the gut—Fig. 5.2 [11].
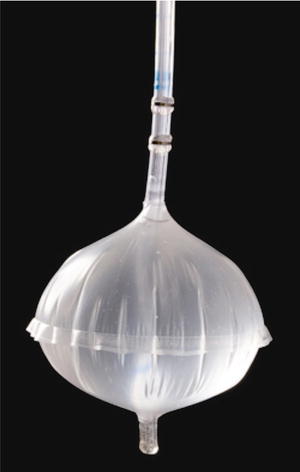
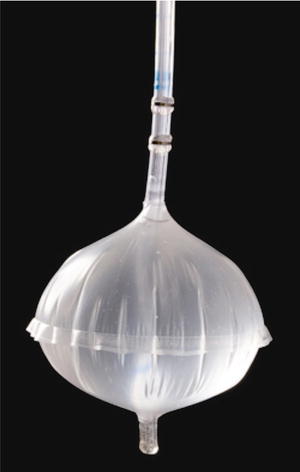
Fig. 5.2
The multimodal probe for electrical, mechanical, cold, warmth, and chemical stimuli. The probe has a bag for mechanical and thermal stimuli, the latter given by recirculating water. Electrodes for electrical stimuli are mounted on the probe above the bag. A side hole in the tube proximal to the bag allows perfusion with acid and other chemicals
Experimental Studies and Pathophysiology of Chronic Abdominal Pain
QST has been used as an attempt to explain the pathophysiology of both functional and organic disorders of the gut. It is generally the belief that the central component of the pain system plays a major role in functional disorders such as functional chest pain, functional dyspepsia, and irritable bowel syndrome (IBS). On the other hand, in organic diseases such as inflammatory bowel disease and chronic pancreatitis, the pain regulatory systems are intact and the balance between afferent activity and local/central pain inhibition is functioning differently. In the following section, selected methods to stimulate and assess the pain system in different examples of functional and organic diseases are highlighted.
In order to unravel abnormal pain processing, several pain assessment methods are available being more or less directly associated with the stimulus. These are for example:
The subjective response using different rating scales
The size and localization of the referred pain area
Detection of viscero-visceral hyperalgesia
The response to repeated stimulation (a proxy to wind-up or central integration)
The subjective sensory response to QST may reflect abnormal processing of the pain. Patients with functional pain disorders, such as functional chest pain and IBS, typically have hyperalgesia and allodynia to experimental stimuli of the organs thought to be diseases [16]. Only 40–60 % of the IBS patients show lowered rectal discomfort thresholds to mechanical stimulation, but when other perceptual abnormalities (altered referral pattern and increased intensity of sensations) were considered, 94 % of IBS patients had at least one abnormality [17]. In order to unravel disease pathogenesis, more advanced methods, such as the multimodal probe, can be used to detect sensory abnormalities. This approach has been used in patients with functional and organic diseases. As stated previously can the TRPV1 receptor be activated by a variety of stimuli, including acid (protons) and increases in temperature that reach the noxious range. Hence, patients with organic diseases, such as nonerosive and erosive esophagitis, were shown to have specific hyperalgesia to heat reflecting activation of the receptor by the natural acid reflux. On the other hand in patients with functional chest pain acid, there was a pathological response to experimental acid perfusion likely reflecting activation of central pain mechanisms [4]. In general, chronic tissue injury and pain has been associated with higher thresholds to mechanical stimulation in different regions of the GI tract. For example, chronic inflammation of the small bowel in patients with inflammatory bowel disease is associated with mechanical hypoalgesia of the rectum [18]. However, the pain response can vary according to the tissues that are stimulated such as seen in patients with chronic pancreatitis. This may reflect the complex pain mechanisms and interaction between sensitization and descending control systems [19, 20]—see also section about viscera-visceral hyperalgesia.
Referred pain is a normal phenomenon seen in clinical practice where pain originating from the viscera is also felt in somatic areas remote from the organ. Convergence between visceral and somatic afferents in the spinal cord seems to be of importance (Fig. 5.3). In organic diseases, it is believed that referred hyperalgesia of somatic tissues is caused by a normal process of central sensitization triggered by massive afferent visceral barrage [21]. However, in functional disorders, abnormal central processing of the afferent stimulation is likely of more importance. Hence, if the patients are properly instructed, the referred pain can be used as a proxy for the central changes. Experimentally, we have found that the referred pain area in healthy volunteers typically changed location after acid perfusion of the esophagus [1–3]. In patients with organic diseases such as those with gastro-esophageal reflux disease (GORD) and chronic pancreatitis, the referred pain area to stimulation of the esophagus and duodenum is increased in size and this is likely reflecting the increased afferent visceral barrage and subsequent activation of second-order neurons [4, 19]. In functional disorders, however, there seems to be a change in localization as well as an increased size of the referred pain area such as seen to experimental visceral stimulation in patients with functional chest pain, functional dyspepsia, and IBS [22].
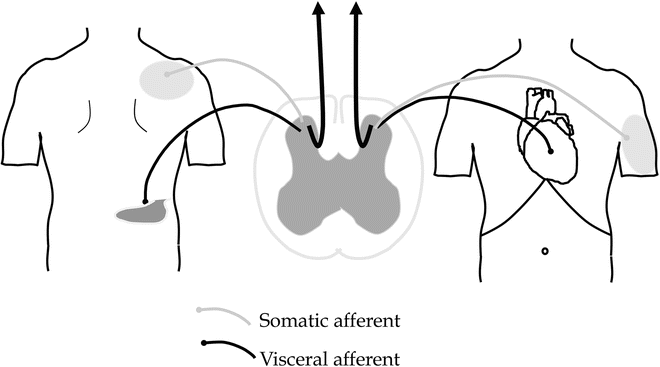
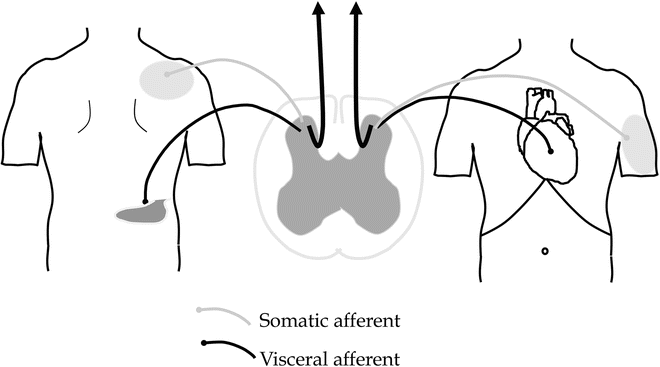
Fig. 5.3
Pain referral to somatic areas remote from the visceral organs is a common finding in GI diseases and known as “referred pain,” e.g., pain referral to the right shoulder in acute cholecystitis. The underlying mechanism is related to convergence between visceral and somatic afferents in the spinal cord although in principal more complicated
Changes in the sensitivity and skin temperature in the referred pain area have also been shown in experimental studies of healthy volunteers [23, 24]. Correspondingly, abnormal superficial and deep sensations have been demonstrated in patients with renal stones, appendicitis, and cholecystolithiasis [25–27]. In patients with chronic pancreatitis sensory changes have also been seen in the corresponding “viscerotome” [28]. Such changes in localization and sensitivity of the referred pain areas may be a hallmark of diseased organs and if the experimental methods are improved they may serve as a biomarker of the disease.
Viscero-visceral hyperalgesia is a complex form of hypersensitivity probably explained by more than one mechanism. Since this phenomenon takes place between visceral organs which share their central afferent termination, it is plausible that central sensitization plays an important role [29]. Recently, human experimental studies support the role of viscero-visceral hyperalgesia in GI diseases. Acidification of the distal esophagus resulted in hyperalgesia in the proximal esophagus, and duodenal acidification was shown to induce esophageal hypersensitivity [30]. Recently, we showed that acidification of the esophagus in healthy volunteers involve widespread changes in the perception of experimental pain from remote organs such as the rectum [31]. The widespread visceral hypersensitivity in functional GI disorders (IBS, functional dyspepsia, etc.) may be due to this mechanism. As an example a marked reduction in colonic perception thresholds and alternation in the viscero-somatic referral pattern were seen in patients with IBS after lipid administration in the duodenum [32]. Viscero-visceral hyperalgesia may also explain the epidemiological findings in several clinical conditions with organic diseases such as an increased number of anginal attacks in patients with gallbladder calcinosis, and increased number of colics in dysmenorrheic patients with urinary calculosis [33]. Evidence for viscero-visceral hyperalgesia has also been provided in experimental studies of organic diseases, e.g., in patients with gastro esophageal reflux disease (GERD) where increased sensitivity to gastric distension was shown. Therefore, the frequent airway symptoms in GERD (often refractory to treatment with proton pump inhibitors) may not only be related to direct aspiration of the gastric refluxate, but vasovagal reflex mechanisms evoked by acid-related hyperalgesia may also be important [34].
Repeated stimulations: Sensitization of the spinal neurons is known to occur with prolonged or repeated stimulation (“wind-up” or temporal summation) of the peripheral afferents. Thus, temporal summation results in a short-lasting spinal cord sensitization that persists after discontinuing the peripheral stimulation. In the laboratory, this is perceived as increased pain to a series of stimuli with the same intensity.
Repeated electrical or mechanical stimuli to the small and large intestine in volunteers may cause increased sensation to subsequent stimuli, and this may be used as a model for enhanced central gain [1–3]. In functional pain Munakata et al. showed the importance of central mechanisms. In their study, patients with IBS developed rectal hyperalgesia following repetitive sigmoid distensions [35]. Paterson et al. [36] as well as studies from our group [4] also showed that repeated distensions conditioned the esophagus in functional chest pain patients resulted in higher pain scores. In organic diseases, repeated stimuli were also used to show the central amplification of pain in patients with chronic pancreatitis [37]. This stimulation paradigm can also be used to understand the changes in referred pain. If electrical stimuli are repeated over time, the pain and the area of referred pain increase progressively [23].
QST can also be used to unravel pain mechanism at higher centers using electrophysiological and imaging methods. There are several possibilities, but the most used methods are as follows:
The nociceptive reflex
Electroencephalography (EEG) and magnetoencephalography (MEG)
Imaging
The nociceptive (RIII) reflex is a spinal reflex that is elicited by painful stimulation of a sensory nerve. For example, stimulation of the sural nerve at the ankle evokes a flexion reflex that can be measured by quantification of the electromyographic response in the biceps femoris muscle. The connection from the primary afferents to the motor neurons is a polysynaptic pathway, which can be modulated by other afferent input, spinal neuronal excitability, and activity in descending control systems. Bouhassira et al. showed that tonic distension of the stomach and rectum resulted in inhibition of the reflex, whereas phasic mechanical stimuli of the rectum resulted in more complex modulation [38, 39]. Sensitization of the esophagus with acid resulted in a significant increase in the baseline reflex excitability, followed by a gradual inhibition during continuous distension of the organ [1–3]. Analgesics can modify the reflex and hence it may indirectly be used for basic and pharmacological studies of pain pathways in the GI tract [40].
The EEG monitors the brain activity to external stimuli directly in real time. The resting EEG has been used to unravel pain mechanisms in visceral diseases [41]. However, when a repetitive stimulus is applied and the cortical electrical activity is averaged (time-locked to the stimulus), the stimulus-evoked cortical potential (EP) can be extracted from the background electrical activity and is shown in shape of a waveform with different peaks (Fig. 5.4). Each peak in the EP represents a synaptic event associated with the transmission of afferent information from one group of neurons to another. The early peaks are supposed only to be influenced by the stimulation rate, intensity, and localization, and they reflect to a major degree the brain loci that process the pain intensity and localization [42]. EPs have been used to explain abnormal central pain processing in patients with functional disorders such as functional chest pain and IBS, suggesting an increased central nervous system response to visceral stimuli and reorganization of brain activation in the cingulate gyrus among others [43–45]. Studies have also suggested that different subgroups of patients with IBS exist such as those with short latency of the early EP components having sensitization of GI afferent pathways, and those with long latencies and enhanced late responses reflecting hypervigilance and increased affective processing [46].
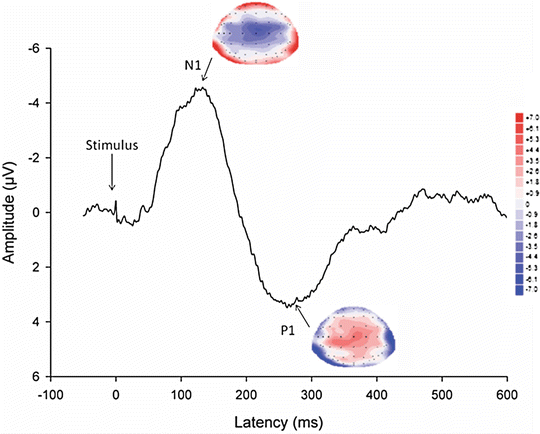
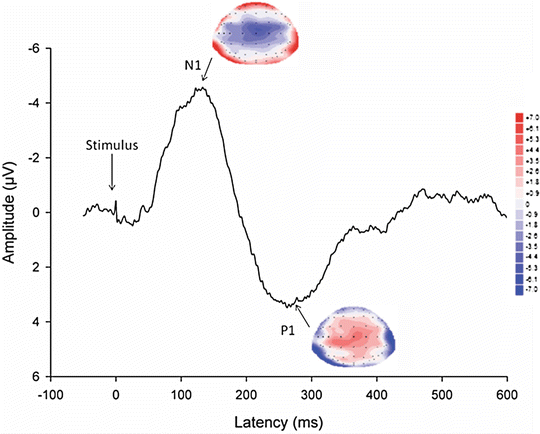
Fig. 5.4
A typical evoked potential (vertex-electrode) recorded after stimulation of the rectosigmoid junction in a healthy volunteer. Note the different peaks denoted N1 and P1, each defined by latency (ms), amplitude (μV), and a corresponding topographic map made from the 64 electrodes covering the head
Inverse modeling of the EPs can be used to identify the original electrical sources in the brain—for details see [47]. In organic diseases such chronic pancreatitis analysis of the EP topography has revealed a shift in insular dipole localization which was correlated with the patients’ clinical pain scores (Fig. 5.5) [48]. Comparable findings have been reported in experimentally induced visceral hypersensitivity in healthy volunteers and may reflect the neurophysiologic correlate of functional reorganization. Insula has an important function for integrating the visceral sensory and motor activity together with limbic integration and is particularly important in pain perception from the gut. Experimental and clinical studies of somatic pain conditions, such as phantom limb pain, have also showed a correlation between clinical pain scores and reorganization, with the most suffering patients showing the most pronounced reorganization (i.e., a maladaptive pain response). Hence, the reorganization in chronic pancreatitis may be due to an “overactivation” of pain areas in the brains pain matrix, inducing a functional reorganization of the insular cortex. Such analysis may increase our understanding of the pain pathogenesis where the pain processing in the brain is of major importance, and there is preliminary evidence that these abnormalities may serve as predictors of treatment response.
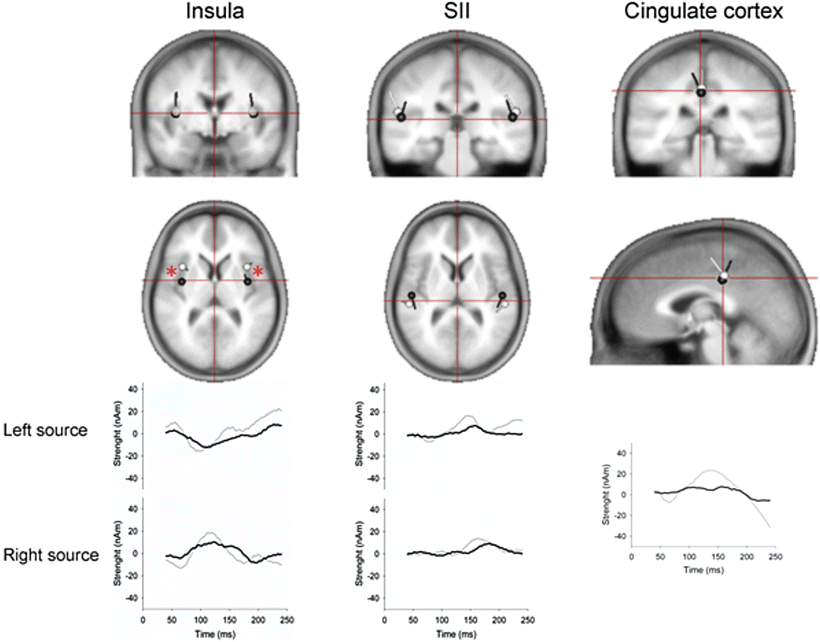
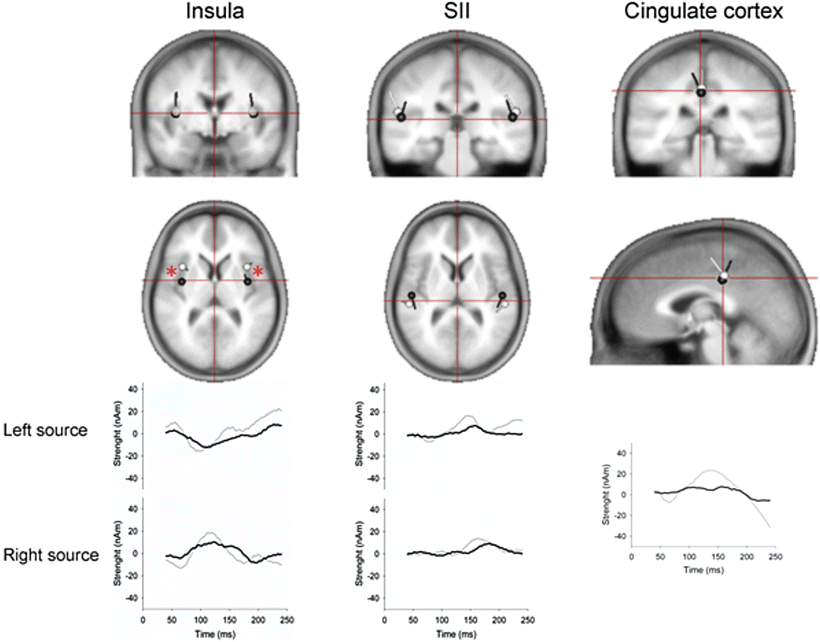
Fig. 5.5
Top panels: locations of brain sources evoked by painful stimulation of the sigmoid in patients with chronic pancreatitis (black) and healthy volunteers (white). The locations of insular sources differed between the groups. Lower panel: sequential activity of the brain sources throughout the time window of analysis (40–240 ms) in chronic pancreatitis patients (black) and healthy volunteers (grey). Modified from Olesen et al. (2011a) [91]
MEG is a noninvasive technique for mapping brain activity by recording magnetic fields produced by electrical currents in the brain. MEG is a technically demanding technique and is only available in few specialist centers. Furthermore, it is limited by its incapability to resolve radial currents generated by deep brain sources, e.g., in the cingulate cortex. However, the spatial resolution of more superficial cortical activity is in the mm range which is better than the EEG (for review see [47]). The methods have been used to follow the brain activation following esophageal electrical stimulation in healthy volunteers, but otherwise studies of visceral pain has until today been very limited [49].
Imaging methods may also be used to explore pain mechanisms following experimental stimulation of the gut. Improved methods for brain imaging techniques (fMRI, PET, and SPECT) have vastly increased our understanding of the central processing of GI sensation and pain in both healthy volunteers as well as in patients suffering from GI disorders.
Magnetic resonance imaging (MRI) allows imaging of both brain structure and activity. Brain activity measured by functional MRI (fMRI) has most commonly been acquired by the blood oxygenation level dependent (BOLD) technique, which is based on different paramagnetic properties of oxy- and deoxyhemoglobin in the blood. fMRI has an excellent spatial resolution (2–5 mm) and operates in a noninvasive and nonradioactive environment allowing subjects to be studied repetitively. The BOLD signal reflects simultaneously changes in local blood flow, volume, and deoxyhemoglobin content, which derive from changes in neuronal activity [50]. Regions of activation are identified by subtracting regional BOLD signal during a control/resting condition from the signal during a stimulus condition—Fig. 5.6. Recently, other techniques such as arterial spin labeling which allows the measurement of whole brain cerebral blood flow in absolute units through the use of magnetically labeled endogenous water in blood allowing assessment of the temporal dynamics of the neural activation induced by pain. This has been used to detect changes in regional cerebral blood flow associated with a standard cutaneous heat pain [49] and infusion of hypertonic saline [51]. Arterial spin labeling is particularly suited to studies of prolonged pain since it becomes increasingly more sensitive than BOLD to changes in neural activation as the stimulus duration exceeds one minute [52]. A new technique called signal enhancement by extravascular water protons has been used in fMRI of the spinal cord, which is essential in the complete mapping of the pain system, and spinal cord and brain stem sensory-related neural activity has been consistently observed in a number of studies. Recently also, resting state fMRI has been applied in pain research including connectivity analysis between multiple brain networks [53]. Additionally, structural information obtained by other MRI techniques can been superimposed on the functional data: diffusion tensor imaging with assessment of microstructural integrity in sensory-related brain areas, tractography with tracing of nerve fibers, volumetry of cortical regions with assessment of the neuroplastic response to long-standing pain, and spectroscopy assessing the concentration of metabolites [54–57]. This allows more explanatory information on the neural structures, function, and connections between the centers involved in pain processing.
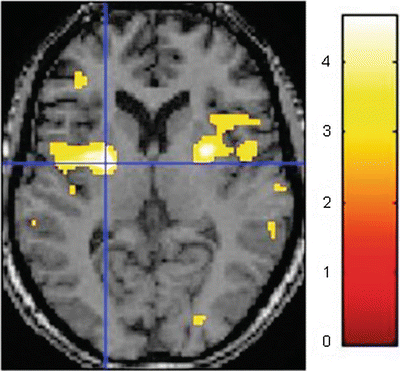
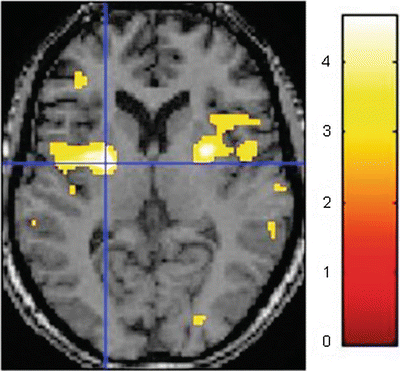
Fig. 5.6
Functional magnetic imaging (fMRI) with illustration of the brain activity induced by painful thermal stimulation of the right forearm in a single subject. This is based on the BOLD technique, which is based on different paramagnetic properties of oxy- and deoxyhemoglobin in the blood where the color code shows signal intensity. Regions of activation (here in the insular regions) are identified by subtracting regional BOLD signal during a resting condition from the signal during the painful stimulus
fMRI has been used in several studies for demonstrating abnormal brain processing in particular functional GI disorders. Few studies have also been conducted in organic diseases such as inflammatory bowel disease. Kwan et al. identified abnormal event-related sensations in five brain regions following rectal distensions in IBS [58]. In the primary somatosensory cortex, urge-related responses in the IBS group were seen compared to the control group. This could be interpreted as upregulated afferent input underlying visceral hypersensitivity or “visceral allodynia.” In the IBS group, pain-related responses were seen in the medial thalamus and hippocampus, but not in the control group. However, pronounced urge- and pain-related activations were present in the right anterior insula and the right anterior cingulate cortex in the control group, but not the IBS group. Finally, lack of activation in right anterior insula was found in IBS patients, interpreted by the authors as either a ceiling effect or a dysfunction in interoceptive processing or control of visceromotor responses. In controls, patients with inflammatory bowel disease and IBS patients, Bernstein et al. performed rectal balloon distention to a sensation of stool and to a sensation of pain while undergoing fMRI [59]. All three groups share similar loci of activations to visceral sensations of stool and pain, but both activation and deactivation of particular regions of interest was differentiated between the groups. Finally, fMRI has been used to evaluate the effect of the tricyclic antidepressant amitriptyline, which is believed to be of clinical benefit in IBS patients [60]. Amitriptyline reduced pain-related cerebral activations in the pACC and the left posterior parietal cortex compared to placebo, but only during mental stress [61].
Single photon emission computed tomography (SPECT) and positron emission tomography (PET) are nuclear imaging techniques that can trace radiolabeled molecules injected into the blood stream, whereby the distribution, density and activity of receptors in the brain can be visualized. This provides an insight into the organization of functional networks in the brain, which cannot be achieved by morphologic investigations or imaging of blood flow and metabolism [62]. Using this molecular imaging technique, pharmaceutical compounds can be used as radiolabeled tracers combined with kinetic models allowing quantification of receptor sites and enzyme function [63]. PET is superior in imaging radiopharmaceuticals and/or other ligands as it offers the ability to study receptor distribution and explore the site of action.
Both SPECT and PET have been used in studies investigating which brain areas are activated during painful stimuli [64]. Nevertheless, it has not been used very widely in clinical pain studies. A study by Fukumoto et al. assessed regional cerebral blood flow of the contralateral thalamus in ten patients with reflex sympathetic dystrophy syndrome [65], but has so far not been used in the investigation of visceral pain. Several studies have used PET for investigating brain activation during visceral pain [66–68], but to our knowledge no studies of specific receptor systems have been conducted.
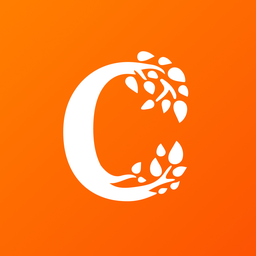
Full access? Get Clinical Tree
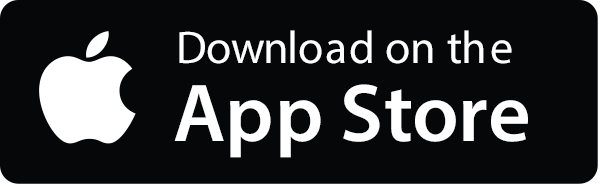
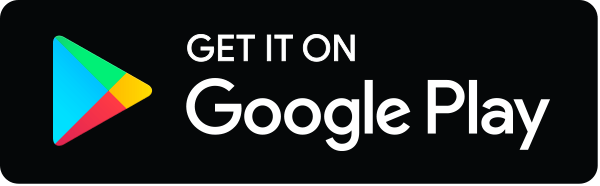