Anabolism is the enzymatic process by which nutrients and energy are used to synthesize molecules in living cells. In contrast, catabolism involves chemical reactions that lead to the degradation of molecules. During growth, anabolic processes dominate, either through increased biosynthesis or decreased molecular degradation. In healthy subjects, energy is produced through the catabolism of carbohydrates and fat, whereas proteins and amino acids are used to generate new structures (i.e., for anabolism). When patients become acutely ill, amino acids from muscle are used to synthesize acute-phase proteins and to generate glucose de novo (i.e., for gluconeogenesis), but this process is limited and adaptive. However, in critical illness, two distinct phases have been described. The first is similar to what is seen in acute illness and is similarly characterized by the diversion of energy and anabolism toward vital organs and the immune system. This response is partially mediated by the endocrine system. Protracted critical illness constitutes the second phase. It is often maladaptive, with uncompensated catabolism leading to major nitrogen loss and muscle wasting, and is characterized by a globally decreased endocrine response. Increased catabolism may be responsible for insufficient wound healing, prolonged mechanical ventilation, and extended hospital lengths of stay. Aggressive nutritional support during this second phase has failed to prevent muscle wasting. It has been hypothesized that hormone supplementation during the protracted phase of critical illness may favor anabolism. Four hormones having anabolic properties may be of interest in the critical care setting: androgens, insulin, growth hormone, and thyroid hormone. The following chapter reviews the evidence regarding the safety and efficacy of anabolic hormone supplementation in critically ill adults.
Androgens
Androgens, or male sex hormones, are synthesized by a series of enzymatic reactions initiated using cholesterol. Cholesterol is first converted into pregnenolone and its metabolite progesterone. These two hormones are converted into 17-hydroxypregnenolone (17-OH-pregnenolone) and 17-hydroxyprogesterone (17-OH-progesterone), respectively, and then into dehydroepiandrosterone (DHEA) and androstenedione. Of note, 17-OH-pregnenolone and 17-OH-progesterone are also precursors of cortisol. DHEA (as well as DHEA-S, the sulfated form of DHEA that predominates in the circulation) and androstenedione, although adrenal androgens, are considered to be largely inactive. Serum DHEA levels are increased during septic shock, whereas those of DHEA-S are decreased. The increase in DHEA concentration may follow the increase in cortisol levels seen during septic shock. DHEA is converted to androstenedione via 3-β-hydroxysteroid dehydrogenase, which is then converted to testosterone by 17-β-hydroxysteroid dehydrogenase. Testosterone can also be converted to estradiol by the aromatase enzyme ( Fig. 71-1 ).

Testosterone is the most abundantly produced and the most clinically relevant androgen. It is secreted by the interstitial cells of Leydig in the testes and by the adrenal glands in males and females. Testosterone secretion is regulated by luteinizing hormone (LH) produced in the anterior pituitary. LH secretion is stimulated by gonadotropin-releasing hormone (GnRH), which is produced by the hypothalamus. Circulating testosterone is bound to albumin or to the sex hormone-binding globulin. Serum concentrations of testosterone are 12 to 31 nmol/L in healthy males and 0.52 to 2.6 nmol/L in healthy females. Testosterone remains in the circulation for no more than a few hours, after which it has either been transported to its target tissues or degraded. At a cellular level, testosterone or its intracellular metabolite, dihydrotestosterone, binds to a nuclear receptor protein complex. This complex migrates to the cell nucleus and induces DNA transcription. Testosterone has well-documented anabolic properties. It induces hypertrophy of type I and II muscle fibers and increases the number of skeletal muscle satellite cells. Testosterone also promotes the differentiation of multipotent mesenchymal cells into myocytes and inhibits their differentiation into adipocytes. Finally, androgens may alter other physiologic parameters via nongenomic signaling pathways that involve lipid and protein metabolism.
Studies in animal models of trauma hemorrhage and sepsis have demonstrated that females have stronger immune responses and better survival rates than males. In the critically ill, though, clinical evidence of an association between female gender and improved outcome is weak and relies on an inconclusive or contradictory literature.
During acute illness, testosterone concentrations are low, whereas LH levels are increased. In patients with prolonged critical illness, serum testosterone, LH, and GnRH concentrations are low. For example, in some patients with chronic obstructive pulmonary disease or human immunodeficiency virus–associated wasting syndromes, administration of synthetic androgens induced a gain in muscle mass and strength and improved respiratory function. In men with severe burn injuries, testosterone reduced protein catabolism. The synthetic androgen oxandrolone, which has been approved by the U.S. Food and Drug Administration as an adjunctive therapy after surgery or trauma, may reduce weight loss, improve functional status, and increase wound healing. However, a large trial involving oxandrolone administration to trauma patients failed to demonstrate any significant benefit. Another trial, performed in ventilator-dependent surgical patients, found that oxandrolone prolonged the duration of mechanical ventilation. Data from trials assessing the effect of testosterone supplementation in critically ill patients on patient-centered outcomes are scarce. Although data are limited, the safety profile of androgens may be favorable. Likewise, data on testosterone precursor (such as DHEA) supplementation in the critically ill are also lacking.
Overall, in the critically ill, androgen supplementation may have some benefits on nutritional endpoints in select subgroups of patients. Additional trials are still needed before the routine use of such a therapeutic approach could be suggested for caring for the critically ill.
Insulin
Insulin is formed by two peptide chains—a 21-amino acid A chain and a 30-amino acid B chain—that are linked by disulfide bonds. The hormone regulates carbohydrate metabolism by promoting glucose entry into cells. Insulin is produced by the beta cells of the islets of Langerhans of the pancreas. The gene for insulin is highly conserved and encodes a single chain precursor. Various factors, including glucose, glucagon, cholecystokinin, and gastric inhibitory polypeptide, induce insulin secretion. Insulin secretion is inhibited by catecholamines and somatostatin. Monomers of insulin, the active form of the hormone, are mostly unbound in the circulation. The half-life of circulating insulin is extremely short, approximately 6 minutes, ensuring that carbohydrate homeostasis occurs almost instantaneously. The fasting serum concentration of insulin ranges from 28 to 108 pmol/L in healthy individuals.
The insulin receptor has strong analogies with the insulin-like growth factor-1 (IGF-1) receptor. Both belong to the receptor tyrosine kinase family. On ligand binding, intracellular portions of the receptor are activated. Activation is followed by the recruitment of adaptor proteins and downstream signaling proteins. One of the downstream signals involves the mitogen-activated protein kinase cascade, which plays a role in modulating cellular proliferation, differentiation, and survival. Insulin receptors can also recruit the insulin receptor substrate adaptor, inducing the activation of phosphoinositide 3-kinase. This enzyme indirectly increases the amount of glucose that is captured by the organism. In addition to its effect on carbohydrate metabolism, insulin also plays a role in protein metabolism and storage, but the mechanics leading to the modification of protein metabolism are still unknown.
Insulin increases the translation of mRNA as well as the rate of transcription of selected DNA genetic sequences. Insulin inhibits the catabolism of proteins, decreases the rate of amino acid release by muscle cells, and stimulates the transport of amino acids into the cells. Insulin inhibits the activity of enzymes promoting gluconeogenesis. Because gluconeogenesis relies on amino acids, insulin indirectly conserves protein and amino acid stores. Insulin promotes protein synthesis in cultured cells, in isolated muscles, and in vivo in animals. A trial conducted in healthy human volunteers, using isotopic tracers, showed that insulin promotes muscle anabolism by stimulating protein synthesis. Reports from trials conducted in burned patients indicate that infusion of insulin improved protein synthesis.
Numerous trials assessing the administration of insulin to general populations of critically ill patients have been conducted in recent years. The aim of these trials was to assess the effect of glucose control on mortality; thus they did not focus on the anabolic effects. A large monocentric trial found that surgical intensive care unit (ICU) patients treated with intensive insulin therapy (target blood glucose levels between 80 and 110 mg/dL) had lower ICU mortality than patients managed with conventional treatment (blood glucose between 180 and 200 mg/dL). A second monocentric trial did not find significant differences in survival rates in medical ICU patients with or without intensive insulin therapy. Subgroup analyses revealed that intensive insulin therapy reduced the mortality rate in patients hospitalized in the ICU for more than 3 days. The Glucontrol multicenter trial compared intensive insulin therapy (target blood glucose between 4.4 and 6.1 mmol/L) with conventional treatment (target blood glucose between 7.8 and 10.0 mmol/L) in the critically ill. In this trial, which was prematurely stopped because of a high number of protocol violations, ICU mortality was similar in both groups. Intensive insulin therapy was associated with an increased incidence of hypoglycemia. The Nice-Sugar trial, including more than 6000 patients, compared intensive insulin therapy (target blood glucose range of 81 to 108 mg/dL), to conventional treatment (target of ≤180 mg/dL). Mortality at day 90 and the incidence of hypoglycemia were significantly higher in the intensive insulin therapy group. The VISEP (Efficacy of Volume Substitution and Insulin Therapy in Severe Sepsis) trial included ICU patients with severe sepsis. In this trial, the mean score for organ failure and the number of deaths at 28 days were not significantly different between the intensive insulin therapy group (target blood glucose levels 80 to 110 mg/dL) and the conventional treatment group (target blood glucose levels 180 to 200 mg/dL). This trial was stopped prematurely because of a significant increase in the incidence of hypoglycemia with the experimental intervention. Lastly, glucose variability might be an independent prognostic factor in critically ill patients.
Current guidelines for the management of sepsis recommend administering insulin to control hyperglycemia. As a general rule, physicians should target a blood glucose level of less than 180 mg/dL during ICU stay. There is no specific recommendation for the administration of insulin as an anabolic hormone.
Growth Hormone
Growth hormone (GH) is structurally similar to prolactin and placental lactogen. Production of GH is positively controlled by the GH releasing hormone (GHRH) and ghrelin. Both are subjected to pulsatile release from the hypothalamus and act on the anterior pituitary to induce pulsatile release of GH. Ghrelin is also produced by the stomach and pancreas. Stress, physical exercise, hypoglycemia, and elevated concentrations of insulin induce the production of GHRH. By contrast, somatostatin, hyperglycemia, obesity, and hypercorticisolism inhibit GHRH production. The serum half-life of GH is between 20 and 30 minutes. The fasting serum concentration of GH in healthy adults is less than 5 ng/mL. GH plays an anabolic role on protein and glucose metabolism, and it stimulates bone growth. The hormone acts either directly through the GH receptors or via the effect of other GHs that have a structure similar to proinsulin and stimulate the uptake of amino acids and inhibit the degradation of muscle proteins. The most important of these hormones is IGF-1, produced by hepatocytes under the influence of GH. IGF-1 is a negative feedback regulator of GH and GHRH. IGF-1 stimulates protein synthesis and decreases the degradation of skeletal muscle proteins. More than 90% of circulating IGF-1 is bound to the IGF binding proteins (IGFBPs).
Critically ill patients have major nitrogen loss associated with muscle wasting. This state has some similarities to that of patients suffering from chronic GH deficiency. The acute phase of a critical illness is characterized by an increased production of pituitary hormones, especially GH, and peripheral resistance to their effects. Overall production of GH is raised through an upsurge in the number and the intensity of pulses and is associated with heightened GH concentrations between pulses and attenuated oscillatory activity. Underlying mechanisms may include increased levels of GHRH and decreased levels of somatostatin. Indeed, in the ICU, elevated GH concentrations seem to be associated with an increased risk of death. In addition, IGF-1 levels are decreased because of the downregulated expression of liver GH receptors or the decreased levels of the main carrier protein IGFBP-3, which is also regulated by GH. These changes are considered adaptive because they could direct the use of glucose, fatty acids, and amino acids toward the production of energy rather than anabolism. During the chronic phase of critical illness, GH and IGF-1 levels decrease even further because of the dramatically reduced pulse amplitude only partially offset by the increased frequency of these same pulses. This neuroendocrine dysfunction seems to be secondary to decreased ghrelin levels. Indeed, high concentrations of ghrelin seem to be associated with a favorable outcome.
IGF-I promotes proliferation and differentiation in muscle cell lines. The administration of GH or of IGF-1 to healthy animals induces muscle hypertrophy. Both GH and IGF-1 administration to animals suffering from burns or major surgery are associated with improved nitrogen balance and immune responsiveness.
Exploratory trials in man show that GH supplementation is associated with a positive nitrogen balance both in patients with and without severe sepsis. One trial reported that GH supplementation after major surgery was associated with a positive nitrogen balance and improved peripheral muscular testing. However, two separate multicenter trials in general ICU populations showed that the administration of recombinant human GH was associated with an increased in-hospital mortality rate. Current guidelines do not recommend the use of GH in the critically ill. The administration of GH releasing peptide-2 (GHRP-2), which is an agonist of ghrelin, is more effective than GHRH for increasing circulating levels of GH, IGF-1, and IGFBP. Additional studies are needed to confirm the efficacy and safety of this treatment. The administration of insulin to the critically ill increases circulating levels of GH and peripheral resistance to GH. Peripheral resistance to GH during protracted illness could be protective.
Opotherapy for GH deficiency is harmful during the acute phase of critical illness. Further studies are needed to examine the effects of GHRH on anabolism during protracted critical illness.
Thyroid Hormones
Thyroid hormones (THs) are synthesized in the thyroid gland. TH synthesis requires the prohormone thyroglobulin and iodine, obtained through normal dietary intake. Tyrosine residues on thyroglobulin can bind iodine once, forming monoiodotyrosine (MIT), or twice, forming diiodotyrosine (DIT). The combination of two DIT residues results in the formation of thyroxine (T 4 ). The combination of one MIT with one DIT residue results in the formation of triiodothyronine (T 3 ) or of the biologically inactive 3,3′,5’-triiodothyronine (reverse T 3 or rT 3 ). These hormones have the same thyronine structure but differ by the number and position of iodine atoms. T 4 is converted by type 1 deiodinase into the more active T 3 . Type 2 deiodinase converts T 4 into T 3 and also converts rT 3 into T 2 (diiodothyronine). Type 3 deiodinase degrades THs, converting T 4 into rT 3 and T 3 into T 2 . The hypothalamic thyroid-releasing hormone (TRH) controls the release of thyroid-stimulating hormone (TSH), which originates in the pituitary gland. In turn, TSH stimulates the production of T 3 and T 4 . TSH has a basal level of constant secretion over which pulses are released. TSH is inhibited by somatostatin and dopamine as well as feedback from T 3 and T 4 . Approximately 80% of THs are transported bound to T 4 -binding globulin, whereas 20% are bound to transthyretin or albumin. Serum concentration of free T 3 is between 4 and 9 pmol/L, whereas the concentration of free T 4 is between 9 and 25 pmol/L and that of TSH is between 0.1 and 4.5 μIU/mL. The half-life of T 4 is 6 to 7 days, whereas that of T 3 is 24 hours. THs enter the cell and bind to nuclear receptors, which mediate the activity of THs via modification of transcriptional activity. THs upregulate all cellular metabolic activities by increasing the number and the activity of mitochondria and accelerating the active transport of ions (potassium, sodium) and glucose across cell membranes. THs also upregulate metabolic activities via nongenomic pathways (i.e., through other means than TH nuclear receptor binding). Physiologic amounts of THs have an anabolic effect and enhance protein synthesis.
In animal models, THs are necessary for anabolism, but supraphysiologicl levels of THs are catabolic. In the ICU, up to 70% of patients have a “nonthyroidal illness syndrome” in which levels of T 3 are diminished and T 4 levels are normal or lowered in the absence of thyroidal disease. TSH is not increased. These anomalies are caused by peripheral mechanisms. Type 1 deiodinase activity is decreased; thus less T 4 is deiodinated into T 3 , whereas the activity of type 3 deiodinase is increased, inactivating THs. The activity of type 2 deiodinase remains almost unchanged during the acute phase of critical illness. Patients also have reduced levels of TH-binding protein, causing lower levels of circulating T 4 . Decreased levels of intracellular transport and of intranuclear receptor expression could be an adaptive mechanism, aimed at increasing tissue levels of the hormones, especially in the liver and the skeletal muscle.
During protracted illness, hormonal disturbances are the consequence of hypothalamic–pituitary axis insufficiency: TRH and TSH levels are low. Decreased TRH secretion could be induced by raised levels of T 3 at the hypothalamic level, either via upregulation of type 2 deiodinase or a downregulation of type 3 deiodinase activity. Consequently, secretion of TSH is also modified. TSH pulsatility is lost, and the amplitude of each pulse is decreased. The pathophysiologic mechanisms explaining these modifications are incompletely understood but may include imbalance in proinflammatory and anti-inflammatory cytokines. However, their exact role remains controversial in animal and human studies.
Clinical trials of TH supplementation were undertaken in several small cohorts. T 4 supplementation increased mortality in patients with acute renal failure. T 3 supplementation increased heart rate and vascular resistances without affecting mortality after major cardiac surgery. The administration of TRH, associated with GHRH or GHRP-2, restored normal TSH and T 3 levels without increasing rT 3 levels. None of these trials showed any clinically relevant beneficial effect of TH supplementation in adults previously devoid of thyroidal disease.
Overall, there is no evidence suggesting any significant benefit from the administration of THs or GHs to the critically ill. Anabolic steroid androgens might improve surrogate outcomes such as weight gain after major surgery or burns. Finally, insulin, which is now broadly given to ICU patients to control blood glucose, may also provide some anabolic effects.
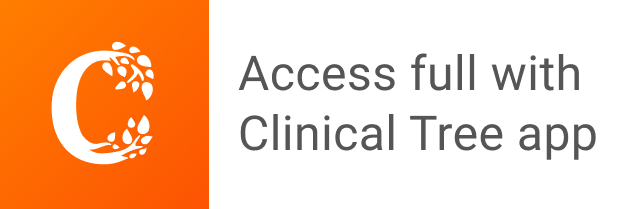