where PR is the pressure required to overcome RAW and PE the pressure required to overcome E. During PPV, the driving pressure for air to flow is applied by the ventilator (PVENT) and, in certain modes, by the patient (PMUS) as well:
where PAPPL is the total pressure applied, either by the respiratory muscles (PMUS), the ventilator (PVENT), or both. PE is determined by the elastance of the respiratory system (E) and the size of the tidal volume (VT):
Using the more familiar entity of compliance (C, the reciprocal of E)
PR is determined by the flow rate of gas (V.) and the RAW
The full interaction between patient and ventilator is described by the equation of motion of the respiratory system (1).
This relatively simple mathematical model has two valuable practical implications: (a) it allows measurement of the patient’s respiratory mechanics at the bedside; and (b) it can be used to predict the independent variable, for example, airway pressure (PAW), for a given value of the set variable (“control”), for example, the VT (2). The first function is widely implemented in modern ventilators: we know the set variable, for example, VT, and the ventilator measures the resulting variable, that is, PAW:
This is accurate if the patient is passively ventilated (PMUS = 0). If the respiratory muscles are contributing to the VT, measuring mechanics as described may not be accurate, because PAPPL is now the result of PVENT and PMUS, but PMUS is unknown. In clinical practice, PMUS can be estimated by measuring the change in intrathoracic pressure in the esophagus (PESO) by means of an esophageal balloon.
The second function forms the basis of the ventilator-control model used in the modern classifications of ventilatory modes (2). The equation shows that for any mode of ventilation only one variable (PAW or V.) can be controlled at one time, that is, on volume-control ventilation (VCV) V. is fixed, so we cannot fix the pressure. VT and V. are very closely related functions: V. is the time derivative of VT, and volume is the time-integral of V., such that we only need to speak about pressure control and volume control.
DESCRIPTION OF A VENTILATOR BREATH
There are three principal components of a ventilator breath: how inspiration begins (trigger), what limits the size of the breath (limit), and how inspiration ends (cycle).
The Trigger
Breaths are triggered either by the patient or by the ventilator (3). If the ventilator initiates the breath, the trigger is time, that is, the operator sets a respiratory rate, and the ventilator will deliver the breath at time intervals to achieve that rate. If the breath is initiated by the patient, inspiration starts when the ventilator detects a pressure or flow change at the airway (pressure trigger and flow trigger), or an electrical signal from diaphragmatic activity in neurally adjusted ventilatory assist (NAVA, later in this chapter).
With pressure trigger (Fig. 104.1) the ventilator senses a decrease of PAW relative to end-expiration, that is, 0 cm H2O or positive end-expiratory pressure (PEEP). A preset decrease of PAW, generally 0.5 to 2 cm H2O, will result in closure of the expiratory valve, opening of the inspiratory valve, and delivery of gas to the airway. With flow trigger, the ventilator senses a change in flow relative to a baseline continuous low flow (bias flow) of gas through the ventilator circuit that most ventilators use in conjunction with flow triggering. A decrease in this bias flow, generally 1 to 3 L/min, will result in initiation of the inspiratory phase. In the special case of NAVA, an electrode array mounted on a special nasogastric feeding tube senses the change in electrical activity of the diaphragm. The change in PAW, V., or electrical signal that triggers inspiration is usually caused by the contraction of the respiratory muscles, but can result from artifacts such as transmission of cardiac oscillations (4), air leaks in the system, or movement of the circuit from water condensate.

FIGURE 104.1 Pressure triggering and flow triggering. With flow triggering, the negative deflection in airway pressure is deeper than flow triggering. This indicates that, if properly set, flow triggering may require less patient work.
In modern ventilators, both flow and pressure triggers are very sensitive (5), and if the trigger sensitivity is set correctly, both modalities are clinically effective (6). If trigger sensitivity is diminished by incorrect setting, failure to trigger spontaneous breaths may increase effort and tax the patient’s reserve. Failure to trigger is often generated by a physiologic problem such as auto-PEEP (7) or respiratory muscle weakness, and requires attention by the clinician to be detected and corrected.
The Limit
The limit variable determines the size of the breath. This is the independent or control variable—that is, the variable set and controlled by the ventilator. Within limits set by alarms and safety mechanisms, this variable is applied independently of the patient’s respiratory mechanics or inspiratory effort. When volume is the preset variable (VCV), the V. and VT are set and the PAW can vary. When pressure is the preset limit variable (pressure-control ventilation [PCV] and pressure support ventilation [PSV]), the PAW is set, and V. and VT are variable.
The Cycle
The cycle variable is what ends the breath. This can be VT, V., PAW, or time. In older ventilators, inspiration was volume-cycled when the volume was delivered from a bellows (Puritan-Bennett MA-1) or piston (Emerson Post-Operative). In modern ventilators, time is the cycle criteria with both VCV and PCV. With PSV, the cycle is usually flow: inspiration ends when the flow rate reaches a fraction of the peak flow. During VCV or PCV, pressure cycle is an alarm condition that avoids application of unsafe high pressure to the airway.
MODES OF VENTILATION
Organization
A mode of ventilation describes the pattern of breathing delivered with a ventilator. Unfortunately, a disproportionate number of proprietary designations exist for a relatively simple structure that is best explained by the equation of motion of the respiratory system Eqs. (6 through 8). That is, (a) for any mode of ventilation only one variable is controlled at one time; even in dual modes, where both pressure and volume may be targeted, this occurs in separate phases of the mechanical breath; and, (b) the result of what is set on the ventilator dependent on the setting itself and the patient’s mechanics, that is, compliance, resistance, and spontaneous effort. The updated classification of ventilator modes describes three basic components: the control variable, the breath sequence, and the targeting scheme (Fig. 104.2). The control variable is what limits the breath, and is discussed in the previous section. Hence, with VCV the PAW varies, and with PCV, the VT varies.
The possible breath sequences are continuous mandatory ventilation (CMV), continuous spontaneous ventilation (CSV), and intermittent mandatory ventilation (IMV). With CMV (also assist/control ventilation [ACV]), every breath is a mandatory breath type, whether initiated from the ventilator or from the patient. With CSV, every breath is a spontaneous breath type. With IMV, spontaneous breathing is allowed between mandatory breaths, so that there will be a mix of mandatory and spontaneous breaths. With CMV or IMV, a minimum rate is set on the ventilator, and the patient can trigger at a more rapid rate. With CSV, there is no set rate other than the alarm parameter (for minute volume or breaths per minute or both) set on the ventilator.
The targeting schemes represent the predetermined goals of ventilator output. The set-point scheme includes most of the traditional modes such as VCV, PCV, and PSV. More complex schemes such as servo and adaptive include proportional assist ventilation (PAV) and volume support (VS) respectively. A complete description of the targeting schemes is available in Table 104.1.

FIGURE 104.2 The taxonomy of ventilator modes: a new classification scheme to describe ventilation modes. (Adapted from Chatburn R. Classification of ventilator modes: update and proposal for implementation. Respir Care. 2007;53(3):323.)
Continuous Mandatory Ventilation
With CMV (also called Assist/Control, A/C) the ventilator supplies full support of the patient’s effort. Disadvantages are the possibilities of hyperventilation and of asynchrony. Hyperventilation (the patient always receives the full breath, even when triggering at a high frequency) is uncommon, because the minute ventilation (VE) is rapidly blunted by the decrease in PaCO2 that follows hyperventilation (8). Asynchrony occurs more frequently, particularly when the level of support is insufficient (see Chapter 105). CMV A/C can be delivered in both volume- and pressure-control modes.
Volume-Control Ventilation
With VCV, the ventilator controls the inspiratory flow and time to deliver the resultant VT. In some cases (e.g., Draeger ventilators) VT, V., and Ti (inspiratory time) are each set. In this case, an inspiratory breath hold occurs if the set inspiratory time is greater than that required to deliver the VT at the selected V.. For example, for a VT of 0.5 L, inspiratory flow of 60 L/min, and inspiratory time 1 second, a 0.5 second breath hold will result. On other ventilators (e.g., Puritan-Bennett 840) an inspiratory hold is set separately.
For VCV, VT, and Ti are the independent variables, and PAW is the dependent variable, as dictated by the equation of motion of the respiratory system Eq. (6), (9,10). Hence, during VCV, the inspiratory pressure applied by the ventilator will increase with a higher VT, higher V., lower C, and higher RAW. Because V. is fixed, a vigorous inspiratory effort will lower the inspiratory pressure, creating asynchrony (9). This can be observed by inspecting the airway pressure waveform (see Chapter 105). In patients with acute respiratory failure, who may have a high respiratory drive, V. should be carefully set to meet the patient’s demand in order to avoid asynchrony and increased (WOB) (11).
TABLE 104.1 Targeting Schemes Used in Current Mechanical Ventilators | ||
![]() |
Volume-control breaths are generally delivered with a constant inspiratory flow waveform, or square wave. On most ventilators, the inspiratory flow can also be set to a descending ramp waveform. With such a flow pattern, the preset peak inspiratory flow is reached early during the breath, after which flow decreases in a linear fashion, reaching a low level at end inspiration (Fig. 104.3). Given the low inspiratory flow at end inspiration, the peak inspiratory pressure (PIP) is lower and approaches the plateau pressure (PPLAT). Note that, in order to accommodate the lower flow and deliver the same VT, the inspiratory time will be longer for a descending ramp than for a constant flow waveform. The longer inspiratory time may result in better oxygenation, but the effect is modest. The longer inspiratory time may also increase the risk or air trapping (auto-PEEP) and hemodynamic compromise.

FIGURE 104.3 Flow, pressure, and volume waveforms during volume-control ventilation with constant flow (left), and descending ramp (right) obtained in a lung model. Note that with the descending ramp flow the peak pressure is lower, the inspiratory time is longer, and, given the low inspiratory flow at end inspiration, the peak inspiratory pressure (PIP) is lower and approaches the plateau pressure (PPLAT). By definition the tidal volume (area under the curve of the flow trace) is unchanged.
The main advantage of VCV is the ability to control the VT. This may be important when the PaCO2 must be closely controlled, such as in patients with traumatic brain injury, or when a low VT is desired as part of a lung-protective ventilation strategy (12,13). Limitations of VCV are related to the fixed VT and inspiratory flow pattern. This can result in asynchrony in actively breathing patients if efforts are not made to set the inspiratory flow appropriately or to provide adequate sedation (14). With VCV, a high PIP may occur with changes in lung mechanics. However, this only increases the risk of lung injury if the high PIP is associated with an increase in plateau pressure (PPLAT). Accordingly, it is important to monitor PPLAT regularly when VCV is used.
Pressure-Control Ventilation
With PCV, inspiratory pressure and time are set on the ventilator. In some cases (e.g., Draeger ventilators), the set pressure is the total inspiratory pressure, but more commonly it is the pressure above PEEP. For PCV, inspiratory pressure and time are the independent variables. The dependent variables are inspiratory V. and VT, as dictated by the equation of motion of the respiratory system Eq. (6). Hence, during PCV, inspiratory V. and VT will increase with a higher set inspiratory pressure, higher C, and lower RAW. Also, V. and VT will increase if the patient generates a vigorous inspiratory effort (i.e., high PMUS). Compared to VCV, this may improve patient-ventilator synchrony (15) but with an increased risk of overdistention lung injury (ventilator-induced lung injury, VILI).

FIGURE 104.4 Flow, pressure, and volume waveforms during pressure-control ventilation. In this instance the inspiratory flow rate reaches 0 before the end of inspiration (blue area). Hence, the airway pressure at end inspiration is a true plateau pressure (PPLAT).
During PCV, the inspiratory flow waveform is a descending ramp (Fig. 104.4). After triggering, the ventilator delivers gas to the airway at a rate dependent on the capability of the ventilator, respiratory mechanics, and patient effort. The set pressure is applied to the airway until the set time is reached. The slope of the descending portion of the inspiratory flow waveform will also depend on the lung mechanics (Fig. 104.5). The initial flow is high, flow descent rapid, and the resulting VT small when C is low, for example, ARDS, pulmonary fibrosis, and large pleural effusions. The initial flow is low, flow descent slow, and VT large when RAW is high, for example, chronic obstructive pulmonary disease (COPD) and asthma.
Many current ventilators allow for the adjustment of the rise time (or pressurization rate), which is the time required to reach the set pressure at the onset of inspiration. A fast rise time (the ventilator reaches the target pressure quickly) is associated with a high flow at the onset of inspiration. A slow rise time (the ventilator reaches the target pressure slowly) is associated with a low flow at the onset of inspiration (Fig 104.6). Patients with a high respiratory drive benefit from a fast rise time; patients with a lower respiratory drive might benefit from a slow rise time (16).
A potential advantage of PCV is that it limits the pressure applied to the alveoli (PALV, clinically estimated by the PPLAT) and the risk of VILI. However, it is important to note that this benefit occurs only if the patient is making no inspiratory effort, because any inspiratory efforts of the patient will increase the transpulmonary distending pressure during PCV. This theoretical advantage of PCV has not been confirmed by appropriately designed clinical trials. The variable inspiratory flow pattern may improve patient–ventilator synchrony during active breathing efforts (15). It seems that in patients receiving small VT during lung-protective strategy, a variable-flow, pressure-targeted breath improves breathing effort compared to a fixed, volume-targeted breath (17).

FIGURE 104.5 Effect of changes in respiratory mechanics on gas flow and tidal volume (VT) during pressure-control ventilation, obtained in a lung model. The left panel was obtained by setting a low compliance: 20 mL/cm H2O, and normal resistance; the resulting VT (the area under the flow curve) was 400 mL. The right panel was obtained by setting a high airway resistance: 20 cm H2O/L/sec and normal compliance; the VT volume was 775 mL. The inspiratory time was 1.5 seconds in both cases; note that with a low compliance (left) the inspiratory flow ends well in advance of the end of the breath, and with a high resistance flow is “cut” by the set inspiratory time, thus limiting the size of the VT.
The square pressure waveform of PCV (and of VCV with a descending ramp flow waveform) produces a higher mean airway pressure than constant flow VCV because the target pressure is reached rapidly and remains at that level through the breath (see Figs. 104.3 and 104.4); this may produce better alveolar recruitment for the same end-inspiratory airway pressure (18). Also, the low end-inspiratory flow with PCV may improve the distribution of ventilation, which may increase PaO2 and decrease PCO2, but the effect is usually modest (19).
A limitation of PCV is the inability to guarantee a VT and a stable PaCO2. With PCV, changes in respiratory mechanics can result in hypoventilation. In particular, PCV can cause hypoventilation in the presence of dynamic hyperinflation and auto-PEEP. For example, if the inspiratory pressure is set at 15 cm H2O and the PEEP is zero, the driving pressure is 15 cm H2O. If the patient develops dynamic hyperinflation and auto-PEEP of 10 cm H2O, the driving pressure is 5 cm H2O, with consequent hypoventilation. With VCV, this will not occur because the set VT is delivered regardless of the level of auto-PEEP, albeit with a higher PIP and PPLAT.
The selection of PCV or VCV is based chiefly on individual preference. If VT, PPLAT, and transpulmonary distending pressure (see Chapter 105) are carefully monitored, either PCV or VCV can be used safely and effectively. Moreover current data from RCTs are insufficient to confirm or refute whether PCV or VCV offers any advantage for patients with acute respiratory failure due to ARDS (20).
Adaptive Pressure Control. Adaptive pressure control allows the ventilator to control pressure based on a volume feedback loop. Because the ventilator controls either pressure or volume, but not both at the same time, there is no divergence from the law of motion of the respiratory system Eq. (6). With adaptive pressure control, the ventilator delivers PCV and adjusts the pressure control in the attempt to keep VT constant.

FIGURE 104.6 Flow and pressure waveforms obtained at increasing rise times of inspiratory flow at a pressure support of 20 cm H2O. The fastest flow delivery (left) results in a rapid reach of the set pressure, thus favoring synchrony for high demand breaths. The slowest delivery (right) may be more comfortable during quiet, unstressed breathing. (Adapted from Gibbons FK, Hess DR. Mechanical ventilation. In: Bigatello LM, ed. Critical Care Handbook of the Massachusetts General Hospital. 4th ed. Philadelphia, PA: Lippincott Williams & Wilkins; 2006.)

FIGURE 104.7 Adaptive pressure control with a set tidal volume of 600 mL. Left: effects of an increase in lung compliance. The first four breaths are at steady state; with the fifth and sixth breaths the tidal volume (VT) is higher due to an increase in compliance as indicated by the steady airway pressure (PAW). From the sixth breath, PAW starts decreasing, and the original VT is restored. Right panel: effects of a decrease in lung compliance. The first four breaths are at steady state; with the fifth breath the VT is lower due to a decrease in compliance as indicated by a steady PAW. From the sixth breath the PAW is increasing, and the original VT is restored. (Adapted from Branson RD, Johannigman JA. The role of ventilator graphics when setting dual-control modes. Respir Care. 2005;50:187, with permission.)
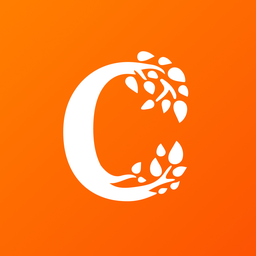
Full access? Get Clinical Tree
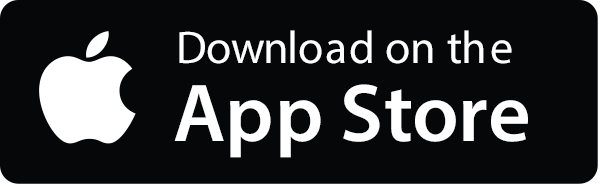
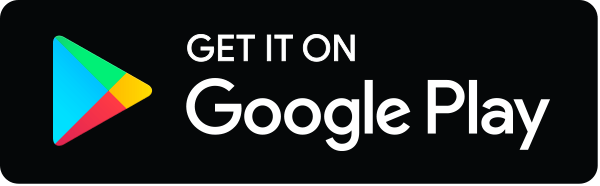