Intravenous Fluid Types
Crystalloids
Crystalloids are fluid solutions containing ion salts and other low-molecular-weight substances. Crystalloids can be categorized based on their tonicity or osmotic pressure of the solution with respect to that of plasma. Examples are listed in Table 17-1. Administering large volumes of normal saline (NS) can result in hyperchloremic metabolic acidosis.4 “Balanced” or “physiologic” crystalloid solutions contain a composition approximating that of extracellular fluid but are usually slightly hypotonic because of lower sodium concentration. Administering large volumes of balanced salt solutions can result in hyperlactatemia, metabolic alkalosis, hypotonicity, and cardiotoxicity due to acetate. Calcium-containing balanced salt solution may cause formation of microthrombi when infused with citrate-containing banked blood.

Colloids
Colloid solutions contain macromolecules suspended in electrolyte solutions. These macromolecules, such as plant or animal polysaccharides or polypeptides, remain in the plasma compartment longer than crystalloid solutions; however, their distribution has been shown to be context sensitive, with larger percentages of the volume administered remaining intravascular in hypovolemic patients as compared to normovolemic patients.3 Semisynthetic colloid solutions are metabolized and excreted and thus have a shorter duration of effect than human albumin solutions.2 Examples are listed in Table 17-2.

Albumin (4% to 5%)
Albumin solution is produced from human blood and suspended in saline. It is heat-pasteurized at 60°C for 10 hours to reduce viral transmission. It is expensive to produce and distribute as compared to semisynthetic colloids and crystalloid solutions. The incidence of anaphylactoid reactions to albumin is 0.011%.5
The comparative effectiveness of fluid resuscitation with colloid versus crystalloid has been a long-standing controversy, which has been the subject of much recent clinical research. In the Saline versus Albumin Fluid Evaluation (SAFE) study, a multicenter, randomized, double-blind trial of 6,997 intensive care unit (ICU) patients, the effect of fluid resuscitation with albumin 4% or NS was evaluated. The primary outcome was death within 28 days. There was no significant increase in mortality (p = .87). The two groups also had similar rates of new single organ and multiple organ failure (p = .85), days spent in ICU (p = .44), days spent in hospital (p = .3), days of mechanical ventilation (p = .74), and days of renal replacement therapy (p = .41).6 In a post hoc analysis of the SAFE study, of 460 patients with traumatic brain injury, the primary outcome of mortality was increased in the albumin-treated group (33.2%) versus the NS group (20.4%, p = .03).7 In an additional subgroup analysis of 1,218 patients with severe sepsis, albumin administration was associated with a decreased risk of death as compared to NS with an adjusted odds ratio of 0.71 (95% CI, 0.52–0.97; p = .03).8 A more recent multicenter, open-label, randomized trial of 1,818 ICU patients with severe sepsis, the Albumin Italian Outcome Sepsis study, of 20% albumin and crystalloid versus crystalloid alone with primary outcome measure of death found no difference in survival at 28 or 90 days (p = .29).9
Semisynthetic Colloid Solutions
Solutions include hydroxyethyl starch (HES) solutions, succinylated gelatin, urea-linked gelatin–polygeline preparations, and dextran solutions. HES, the most commonly used semisynthetic colloid solutions, are created by attaching hydroxyethyl groups to carbons 2, 3, or 6 of the glucose moieties of starches of sorghum, maize, or potatoes. HES solutions vary with respect to HES concentrations (6% to 10%), molecular weights (70 to 670 kDa), molar substitution ratios (0.3 to 0.75), and crystalloid carrier solutions. The concentration influences the initial volume effect with 6% solutions being iso-oncotic and 10% solutions hyperoncotic. HES are polydisperse with particles in a wide range of molecular mass (dispersity is a measure of the heterogeneity of sizes of molecules or particles in a mixture); thus, the molecular weight is averaged by either weight or number, with high molecular weight preparations being associated with alterations in coagulation. The substitution ratio indicates the average fraction of glucose moieties bearing a hydroxyethyl group. HES can also be named hexa- (0.6), penta- (0.5), or tetra- (0.4) starches for this level of substitution. Substitution increases the solubility of the starch in water and inhibits the destruction of the starch by amylase, thus prolonging intravascular retention. HES can also be categorized with respect to the pattern of hydroxyethylation of the C2 and C6 carbon atoms. Hydroxyethyl groups in the C2 position inhibit amylase access to the starch more effectively than hydroxyethyl groups at the C6 position; thus, high C2/C6 ratios would be expected to hydrolyze more slowly. The maximum daily dose of HES is limited to 20 to 50 mL/kg of body weight/day but varies by solution.10
HES is removed from the circulation by redistribution and renal excretion. Redistribution of HES results in temporary storage in the skin, liver, and kidneys. Skin deposition results in non–histamine-associated pruritus. After 24 hours, 23% of the total dose is interstitial and at 26 weeks, trace amounts of HES are still detectable.6 HES molecules with greater molecular weights and increased substitution ratios tend to be stored more than those with more rapid clearance and deposition appears to be dose-dependent.10
Renal excretion of HES occurs in two phases: immediate glomerular filtration of HES polymers less than 59 kDa and delayed glomerular filtration after HES metabolism by plasma α-amylase. This amylase functions as an endoamylase cleaving within the polyglucose chain instead of acting at the ends of the molecule, resulting in polydispersity and varying molecular weights of the remaining HES molecules in the plasma. Thus, pharmacokinetic parameters of plasma clearance and half-life will change over time, cannot be rigorously defined, and must not be interpreted as efficacy half-lives or contributing to the pharmacodynamics of volume effect of HES solutions.11 Additionally, the hydroxyethyl groups retard hydrolysis of the compound by amylases, allowing longer presence in the plasma. Plasma levels of amylase are elevated after HES administration for 72 hours, without evidence of increased pancreatic production, owing to decreased renal elimination of amylase as it remains complexed to HES.5 The pharmacokinetic profile of some HES solutions after single dose and multiple infusions in healthy volunteers is described in Tables 17-311 and 17-4,11 and in impaired renal function in Table 17-5.12



HES compounds have effects on coagulation with reductions in factor VIII, von Willebrand factor, and platelet function, although the exact mechanisms are unclear. Coagulation effects are noted even when used below recommended maximum doses. Solutions with more rapid degradation are associated with less effects on coagulation.10 The incidence of anaphylactoid reactions with HES use is 0.085%.6
HES solutions carry a U.S. Food and Drug Administration black box warning with the following recommendations: Do not use HES solutions in critically ill adult patients including those with sepsis and those admitted to the ICU; avoid use in patients with preexisting renal dysfunction; discontinue use of HES at the first sign of renal injury; need for renal replacement therapy has been reported up to 90 days after HES administration, continue to monitor renal function for at least 90 days in all patients; avoid use in patients undergoing open heart surgery in association with cardiopulmonary bypass due to excess bleeding; discontinue use of HES at first sign of coagulopathy.13
The Crystalloid versus Hydroxyethyl Starch Trial evaluated HES versus NS in a multicenter, prospective, blinded, parallel-group, randomized controlled trial of over 7,000 adult ICU patients.14 Patients were randomized to receive either HES (6% [130/0.4] Voluven, Fresenius Kabi Norge AS, Halden, Norway) solution or NS until ICU discharge, death, or 90 days following randomization. Primary outcome was death 90 days after randomization, and secondary outcomes were acute kidney injury, failure, and treatment with renal replacement therapy. There was no significant difference in mortality during the study period (18% in the HES group and 17% in the NS group, p = .26) or renal failure (HES group 10.4% and 9.2% NS group, p = .12); however, significant differences in renal injury (34.6% HES group and 38% NS group, p = .005) and renal replacement therapy use (7% HES group, 5.8% NS group, p = .04) were found. Additionally, HES was associated with significantly more adverse events (0.3% vs. 2.8%, p <.001).
HES (6% [130/0.42] Tetraspan, B. Braun Melsungen AG, Melsungen, Germany) has also been evaluated in a multicenter, parallel-group, blinded, randomized trial of 798 adult ICU patients with severe sepsis versus Ringer’s acetate in the Scandinavian Starch for Severe Sepsis/Septic Shock trial. Primary outcomes measured were death or end-stage kidney failure at 90 days. Death was greater at 90 days in the HES group (51% vs. 43%, p = .03). One patient in each group had end-stage kidney failure; however, in the 90-day period, 22% of HES patients were treated with renal replacement therapy versus 16% in the Ringer’s acetate group (p = .04).15
In the Efficacy of Volume Substitution and Insulin Therapy in Severe Sepsis multicenter, randomized trial evaluating adult ICU patients with severe sepsis, patients were randomized to receive either intensive insulin therapy or conventional insulin therapy in addition to either HES 10% pentastarch, HES 200/0.5, or lactated Ringer’s for fluid resuscitation. Primary endpoints were death and mean score for organ failure. There were 537 patients who were evaluated and the trial was stopped early due to increased severe hypoglycemia events in the intensive insulin therapy group, but the comparison between HES and lactated Ringer’s was continued with all patients receiving conventional insulin therapy. HES therapy was associated with higher rates of acute renal failure (34.9%, 22.8% in the lactated Ringer’s group, p = 0.002) and renal replacement therapy than lactated Ringer’s (18.3%, 9.2% lactated Ringer’s group).16
Assessing Fluid Responsiveness
Fluid responsiveness may be defined as a 15% increase in cardiac output following a 500-mL IV fluid bolus, indicating that the patient is still on the ascending limb of the cardiac output/end diastolic volume curve, also referred to as the cardiac function curve17 (Fig. 17-2). Fluid administration to a patient on the plateau part of the curve may be of little benefit and result in adverse effects. Filling pressure measures, particularly central venous pressure, correlate poorly with blood volume, and changes in central venous pressure have been shown to poorly predict hemodynamic response to fluid challenge.18 Stroke volume changes due to increases or decreases in right ventricular preload may be used to assess fluid responsiveness. Positive pressure ventilation decreases right ventricular stroke volume by decreasing venous return to the right heart and increasing right ventricular afterload. The decrease in right ventricular stroke volume is passed on to the left ventricle over subsequent cardiac cycles and if the left ventricle is preload dependent, decreases in the left ventricle stroke volume will cause a decrease in the arterial pulse pressure. These cyclic changes associated with positive airway pressure are greater when the ventricles are functioning on the steep, ascending portion of the cardiac function curve. Variation in the arterial pulse pressure (PPV) can be derived from analysis of the arterial pressure waveform and variation greater than 12% to 13% is predictive of volume responsiveness.19 Other measures of these positive pressure–associated stroke volume changes include systolic pressure variation of the arterial waveform, stroke volume variation (SVV) derived from arterial pulse contour analysis, pleth variability index derived from pulse oximeter waveform analysis, inferior vena cava diameter variation measured by echocardiography, and descending aortic blood velocity measured by esophageal Doppler. Measures dependent on variations caused by positive pressure ventilation are limited by the presence of arrhythmia, spontaneous breathing, tidal volume settings (8 mL/kg ideal body weight minimum required for PPV and SVV),19 low lung compliance (<30 mL/cm H2O), increased abdominal pressure, and open chest surgery.20

The end-expiratory occlusion test is useful in ventilated patients with cardiac arrhythmias, mild amplitude spontaneous breathing activity, or low tidal volume positive pressure ventilation. The test assesses the effect of a 15-second interruption in the ventilation on cardiac preload. A 5% increase in pulse contour cardiac output (sensitivity 91%, specificity 100%) or pulse pressure (sensitivity 87%, specificity 100%) is suggestive of volume responsiveness.21 Passive leg raising maneuvers (PLR) can be used to assess preload responsiveness in spontaneously breathing patients with arrhythmias but is limited in patients with intraabdominal hypertension. The test is performed in a supine patient by elevating the legs to 45 degrees while assessing cardiac output or stroke volume over 30 to 90 seconds. Cardiac output measures during PLR are more accurate in predicting fluid responsiveness than arterial pressure measurements during the maneuver.22
Important Fluid Constituents
Magnesium
Magnesium is almost all intracellular in bone (53%), muscle (27%), and soft tissues (19%), with less than 1% of total body magnesium in the extracellular fluid and only 0.3% in the plasma.23 Most intracellular magnesium is bound to adenosine 5’-triphosphate and DNA, with less than 3% being in solution and ionized immediately available for intracellular magnesium homeostasis.24 Plasma magnesium level is normally 1.7 to 2.4 mg/dL, where it is found in three states: ionized (62%), protein bound (33%), and complexed to anions (5%).23 Given these distributions, plasma magnesium measurements may not be representative of total body magnesium stores. Also, magnesium measurements can be falsely elevated with hemolysis of the blood sample, which releases the intracellular electrolytes. Ingested magnesium is absorbed in the small intestines, primarily the ileum (75%),24 via passive concentration effects and in the colon by active transcellular absorption.25 Excretion occurs via the kidney with more than 95% of the filtered magnesium being reabsorbed in the renal tubules, with this mechanism effectively regulating the plasma level. Reabsorption occurs primarily (70%) in the ascending loop of Henle via passive mechanisms with a small amount occurring in both the proximal tubule via passive mechanisms and distal convoluted tubules via active mechanisms.24 Bone, as the primary store of total body magnesium, provides a buffer for plasma magnesium levels through poorly understood mechanisms controlling magnesium incorporation into bone by osteoblasts and removal by osteoclasts.25 Genetic mutations in colon transport channels25 and loop of Henle junction proteins24 can both result in hypomagnesemia.
Role of Magnesium
Magnesium plays a key role in many biologic processes including protein synthesis, neuromuscular function, and nucleic acid stability. It is involved in adenosine 5′-triphosphatase function, antagonizes N-methyl-D-aspartate (NMDA) glutamate receptors, inhibits catecholamine release, and is involved in the regulation of other electrolytes. For instance, magnesium antagonizes the uptake and distribution of calcium and modulates sodium and potassium currents thru nicotinic acetylcholine receptors, NMDA receptors, and ion pumps, thus affecting membrane potentials. Magnesium has antiarrhythmic properties related to calcium channel antagonism.24 Intravenous (IV) magnesium administration can exert muscle-relaxing effects, enhance nondepolarizing neuromuscular blockers, attenuate muscle fasciculations and potassium release with administration of succinylcholine, and precipitate skeletal muscle weakness in patients with Lambert-Eaton syndrome and myasthenia gravis. It has been used to reduce anesthetic requirements and attenuate cardiovascular effects of laryngoscopy and intubation. Magnesium has been shown to vasodilate blood vessels in many vascular beds (mesenteric, skeletal muscle, uterine, cerebral, coronary, and the aorta). It also decreases blood–brain barrier disruption and limits cerebral edema formation after brain injury.26 Side effects of IV administration include burning or pain on injection, drowsiness, nausea, headache, dizziness, muscle weakness, hypotension, and bradycardia.
Hypomagnesemia
Hypomagnesemia may result from dietary deficiency (as seen in chronic alcoholism), gastrointestinal malabsorption or secretion (diarrhea, vomiting, laxative use), renal losses (medication effects, nephrotoxic agents, endocrine disease, diabetic nephropathy), and chelation (citrate binding in the case of massive transfusion).24 It is seen in as many as 11% of hospitalized patients and 65% of patients in the ICU. Clinical manifestations of hypomagnesemia result in cardiac and neuromuscular disorders and include symptoms of nausea, vomiting, weakness, convulsions, tetany, fasciculations, as well as electrocardiogram (ECG) abnormalities (prolonged PR and QT intervals, diminished T-wave morphology, torsades de pointes, and others) and accompanying hypokalemia and hypocalcemia.
Hypermagnesemia
Hypermagnesemia is rare and most commonly occurs with excessive administration of magnesium for therapeutic purposes. Clinical manifestations include QRS widening, hypotension, narcosis, diminution of deep tendon reflexes, respiratory depression from paralysis of muscles of ventilation, heart block, and cardiac arrest. Immediate treatment of life-threatening hypermagnesemia is with calcium gluconate, 10 to 15 mg/kg IV, followed by diuretics or dialysis, along with appropriate respiratory and circulatory support.
Preeclampsia
Magnesium appears to improve the clinical symptoms of preeclampsia by causing systemic, vertebral, and uterine vasodilation via direct effects on vessels as well as by increasing concentrations of endogenous vasodilators (endothelium-derived relaxing factor and calcitonin gene–related peptide) and attenuating endogenous vasoconstrictors (endothelin-1). Suggested dosing regimens of magnesium sulfate based on randomized trial data are 4 g IV loading dose over 10 to 15 minutes followed by infusion of 1 g per hour for 24 hours or 4 g IV loading dose with 10 g intramuscular (IM) followed by 5 g IM every 4 hours for 24 hours. Many other dosing regimens exist.27 Infusions or repeat dosing should be combined with clinical monitoring of urine output, respiratory rate, and deep tendon reflexes. Serum monitoring of magnesium levels should be performed for signs of toxicity or renal impairment. Magnesium crosses the placenta and may result in neonatal lethargy, hypotension, and respiratory depression if administered for prolonged duration (more than 48 hours).24 In a Cochrane Summaries review, magnesium was shown to decrease the risk of progression to eclampsia (RR, 0.41; CI, 0.29–0.58), decrease the risk of placental abruption (RR, 0.64; CI, 0.5–0.83), and increase caesarean section (RR, 1.05; CI, 1.01–1.1) but does not clearly affect maternal morbidity, stillbirth, or neonatal death or neurosensory disability at age 18 months. Reductions in maternal death were found to be nonsignificant.28
Cardiac Dysrhythmias
Excess magnesium blocks myocardial calcium influx resulting in decreased sinus node activity, prolonged atrioventricular (AV) conduction time, and increased AV node refractoriness. Arrhythmias associated with hypomagnesemia are often29 accompanied by hypokalemia. Normalization of both electrolytes is recommended.23 Magnesium administration may decrease the incidence of severe arrhythmia after myocardial infarction but use is limited by the incidence of hypotension.24 There is no evidence that magnesium infusion during human cardiopulmonary resuscitation increases survival to hospital discharge; however, magnesium is recommended for patients with polymorphic wide complex tachycardia associated with familial or acquired long QT syndrome (torsades de pointes).30 For digoxin-induced tachyarrhythmias in hypomagnesemic patients, magnesium should be administered while awaiting digoxin antibodies.24 Prophylactic administration of magnesium during cardiopulmonary bypass has been shown to decrease the incidence of postoperative atrial fibrillation after coronary artery bypass graft surgery.
Analgesia
Magnesium has antinociceptive effects when administered IV or intrathecally, possibly due to inhibition of calcium influx, antagonism of NMDA receptors, or prevention of NMDA signaling. Data to support the use of magnesium as an analgesic or for preventative analgesia at this point is conflicting.24
Asthma
Magnesium causes bronchodilatation via inhibition of calcium-mediated smooth muscle contraction, inhibition of histamine release from mast cells, and inhibition of nicotinic acetylcholine release. IV magnesium (not inhaled) has been reported to improve bronchodilatation when standard therapies have failed; however, responses are variable.23,31
Pheochromocytoma
Magnesium’s arteriolar-dilating effects combined with reduction in catecholamine release may be beneficial in the management of patients with pheochromocytoma prior to tumor excision and in hemodynamic catecholamine crisis.32,33
Calcium
As an important component of the skeleton, there is more calcium in the body than any other mineral. The plasma concentration of calcium is maintained between 4.5 and 5.5 mEq/L (8.5 to 10.5 mg/dL) by an endocrine control system involving vitamin D, parathyroid hormone, and calcitonin, which regulate intestinal absorption, renal reabsorption, and bone turnover. Total plasma calcium consists of calcium bound to albumin and proteins (40%), calcium complexed with citrate and phosphorus ions (9%), and freely diffusible ionized calcium (51%).34 It is the ionized fraction of calcium that produces physiologic effects and is normally 2 to 2.5 mEq/L. The ionized concentration of calcium depends on arterial pH, with acidosis increasing and alkalosis decreasing the concentration. Additionally, plasma albumin binds nonionized calcium, thus, in low albumin states, less nonionized calcium is protein bound making more available to return to storage sites, such as bone and teeth. This may decrease the total plasma calcium, but symptoms of hypocalcemia do not occur unless the ionized calcium concentration is also decreased. Thus, nonionized plasma calcium levels must be interpreted with knowledge of the plasma albumin concentration and can be corrected according to the following formula: corrected Ca++ (mg/dL) = measured Ca++ (mg/dL) + [0.8 × (4.0 − albumin (mg/dL)].35 However, calculations to correct serum nonionized calcium for hypoalbuminemia may not be reliable in critically ill patients.36
Role of Calcium
The majority of total body calcium (>99%) is present in bone and provides the skeleton with strength and a reservoir to maintain the intracellular and extracellular calcium concentrations. Calcium is important for neuromuscular transmission, skeletal muscle contraction, cardiac muscle contractility, blood coagulation, and intracellular signaling in its function as a second messenger. In cardiac myocytes, calcium regulates contraction, relaxation, and electrical signals that determine rhythm and triggers hypertrophy via calcineurin mechanisms.37 In vascular smooth muscle, calcium induces a change in contractile state, increasing and decreasing vessel diameter.38
Hypocalcemia
Hypocalcemia can result from decreased plasma concentration of albumin, hypoparathyroidism, acute pancreatitis, vitamin D deficiency, chronic renal failure associated with hyperphosphatemia, citrate binding of calcium (in the case of transfused blood, particularly in hepatic failure and reduced citrate metabolism39,40 or use of citrate in dialysis or plasmapheresis41), sepsis, and critical illness.41 Malabsorptive states rarely result in hypocalcemia as serum levels are maintained by bone calcium stores. Symptoms of hypocalcemia include neuromuscular excitability, including muscle twitching, spasms, tingling, numbness, carpopedal spasm, tetany, seizures, and cardiac dysrhythmias.42 Calcium can be administered by oral or IV route. IV preparations include calcium chloride which provides 27 mg of elemental calcium/mL and calcium gluconate which provides 9 mg.41 IV calcium chloride may cause local irritation and necrosis if extravasated into the subcutaneous tissues and therefore is best administered centrally.
Hypercalcemia
Hyperparathyroidism is the most important cause of hypercalcemia and may be primary from parathyroid adenoma (85%), parathyroid hyperplasia (10%) which may be associated with multiple endocrine neoplasia syndromes, or, rarely (<1%), parathyroid carcinoma. Secondary hyperparathyroidism results from abnormal feedback loops present in renal failure and tertiary hyperparathyroidism from overactive responses to normal negative feedback mechanisms. Malignancies, such as squamous cell lung, breast, prostate, colon, adult T-cell, and multiple myeloma, may result in release of parathyroid hormone–related peptide from tumor cells, resulting in inappropriate hypercalcemia.41 Malignancy-related hypercalcemia may also result from osteolytic activity at sites of skeletal metastases commonly seen in breast cancer, multiple myeloma, and lymphoma, and, rarely, malignancy-related hypercalcemia may result from tumor release of vitamin D.35 Hypercalcemia may be associated with benign familial hypocalciuric hypercalcemia syndrome resulting from a mutation in calcium-sensing receptors. Hypercalcemia is also associated with granulomatous diseases such as sarcoidosis, tuberculosis, leprosy, coccidioidomycosis, and histoplasmosis and may result from excessive dietary supplement or medication side effects as a result of diuretic or lithium administration. Symptoms of hypercalcemia result from smooth muscle relaxation in the gut (constipation, anorexia, nausea, vomiting), decreased neuromuscular transmission (lethargy, hypotonia, confusion), renal effects (polyuria, dehydration, nephrolithiasis), cardiac rhythm abnormalities (QTc shortening, J waves following QRS complex), as well as pancreatitis.41
Treatment of hypercalcemia depends on the exact etiology but usually includes promoting renal excretion of calcium with IV fluids and loop diuretics while avoiding dehydration that would worsen any renal injury. Medications contributing to hypercalcemia should be discontinued and parathyroidectomy performed if indicated. Corticosteroids can be used to lower excessive calcium levels by inhibiting the effects of vitamin D, reducing intestinal absorption, and increasing renal excretion. Hydrocortisone 200 to 400 mg IV per day for 3 to 5 days41 or prednisone 40 to 100 mg per day orally are recommended treatments for hypercalcemia associated with lymphoma and myeloma.35 IV bisphosphonates to inhibit osteoclast bone resorption may be useful: pamidronate 60 to 90 mg IV or zoledronate 4 mg IV. Gallium nitrate 100 to 200 mg/mL/day IV infusion for 5 days is used to inhibit osteoclastic bone resorption for paraneoplastic hypercalcemia refractory to bisphosphonate therapy.35 Calcitonin 4 to 8 International Units/kg subcutaneously or IM every 12 hours is less effective than bisphosphonates35 or gallium nitrate41 and works by inhibiting bone resorption and increasing renal calcium excretion. Mithramycin 25 µg/kg IV blocks bone resorption by inhibiting osteoclast RNA synthesis, but its use is limited by frequent dosing and toxicity (renal, hepatic, and hematologic).35 Hemodialysis may also be used to treat acute, severe hypercalcemia.
Bone Composition
Bone is composed of an organic matrix that is strengthened by deposits of calcium salts. The organic matrix is greater than 90% collagen fibers, and the remainder is a homogeneous material called ground substance. Ground substance is composed of proteoglycans that include chondroitin sulfate and hyaluronic acid. Salts deposited in the organic matrix of bone are composed principally of calcium and phosphate ions in a combination known as hydroxyapatites.
The initial stage of bone production is the secretion by osteoclasts of collagen and ground substance. Calcium salts precipitate on the surfaces of collagen fibers, forming nidi that grow into hydroxyapatite crystals. Bone is continually being deposited by osteoblasts and is constantly being absorbed where osteoclasts are active. The bone-absorptive activity of osteoclasts is regulated by the parathyroid gland. Except in growing bones, the rate of bone deposition and absorption are equal, so the total mass of bone remains constant.
Because physical stress stimulates new bone formation, calcium is deposited by the osteoblasts in proportion to the compression load that the bone must carry. The deposition of bone at points of compression may be caused by small electrical currents induced by stress, called the piezoelectric effect, stimulating osteoblastic activity at the negative end of current flow. Osteoblasts are maximally activated at a bone fracture, the resulting bulge of osteoblastic tissue and new bone matrix being known as callus.
Osteoblasts secrete large amounts of alkaline phosphatase when they are actively depositing bone matrix. As a result, the rate of new bone formation is reflected by elevation of plasma concentration in alkaline phosphatase. Alkaline phosphatase concentrations are also increased by any disease process that causes destruction of bone (e.g., metastatic cancer, osteomalacia, and rickets).
Calcium salts almost never precipitate in normal tissues other than bone. A notable exception, however, is atherosclerosis, in which calcium precipitates in the walls of large arteries. Calcium salts are also frequently deposited in degenerating tissues or in old blood clots.
Bisphosphonates
Bisphosphonates are drugs with a phosphorus-carbon-phosphorus (P-C-P) chemical structure that resemble inorganic pyrophosphate (Fig. 17-3).43 Inorganic pyrophosphate is involved in regulation of bone mineralization by binding hydroxyapatite crystals, inhibiting calcification. The phosphate groups of bisphosphonates, like inorganic pyrophosphate, bind hydroxyapatite crystals and become incorporated into sites of active bone remodeling, thus inhibiting calcification. The hydroxyl group attached to the central carbon further increases bisphosphonate’s ability to bind calcium, and the final structural grouping is attached to the central carbon to determine the bisphosphonate’s potency for inhibition of bone resorption. First-generation bisphosphonates (etidronate, clodronate, tiludronate), similar to inorganic pyrophosphate, become incorporated into adenosine triphosphate (ATP) by class II aminoacyl-transfer RNA synthetases after osteoclast-mediated uptake from bone and mineral surface. This abnormal ATP cannot be hydrolyzed, accumulates, and is believed to be cytotoxic to osteoclasts. Second- and third-generation bisphosphonates (alendronate, risedronate, ibandronate, pamidronate, and zoledronic acid) contain nitrogen or amino groups in this position, which increases the antiresorptive potency by binding and inhibiting farnesyl pyrophosphate synthase, leading to osteoclast apoptosis. Second- and third-generation bisphosphonate–induced osteoclast apoptosis can be detected by a reduction in biochemical markers of bone resorption; maximum suppression occurs within 3 months of initiation of oral therapy. Suppression is noted to be more rapid following IV administration. Duration of effect is a function of potency for mineral matrix binding, with zolendronic acid suppressing biochemical markers of bone resorption for up to 1 year in women with postmenopausal osteoporosis.

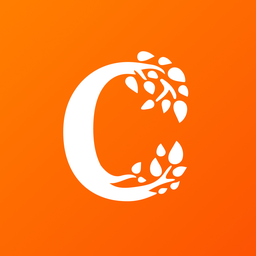
Full access? Get Clinical Tree
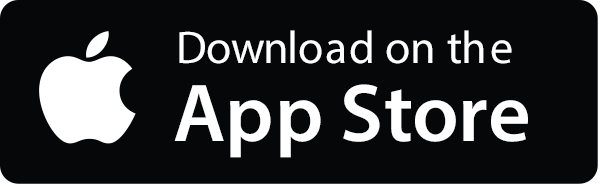
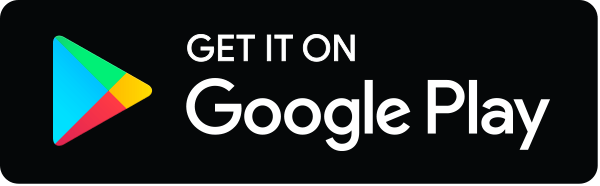