Key Points
- ▪
Anesthesia consists of separable and independent components, each of which involves distinct, but possibly overlapping, mechanisms at different sites in the central nervous system.
- ▪
The potencies of general anesthetics correlate with their solubility in oil, indicating the importance of interactions with predominantly hydrophobic targets.
- ▪
General anesthetics act by binding directly to amphiphilic cavities in proteins. Binding sites have been identified by a combination of site-directed mutagenesis and high-resolution structural analysis of anesthetic binding.
- ▪
Mutations made to render putative protein targets insensitive to inhaled anesthetics have been expressed in mice but have not generated breakthroughs analogous to the success of this strategy with intravenous anesthetics.
- ▪
The effects of inhaled anesthetics cannot be explained by a single molecular mechanism. Rather, several targets contribute to the component actions comprising the anesthetic effects of each anesthetic. However, these effects do converge on a limited number of states underlying the behavioral effects.
- ▪
The immobilizing effect of inhaled anesthetics involves actions in the spinal cord, whereas sedation/hypnosis and amnesia involve supraspinal mechanisms that interact with endogenous memory, sleep, and consciousness pathways and networks.
- ▪
Volatile inhaled anesthetics enhance inhibitory synaptic transmission postsynaptically by potentiating ligand-gated ion channels activated by γ-aminobutyric acid (GABA) and glycine, extrasynaptically by enhancing GABA receptors, and presynaptically by enhancing basal GABA release.
- ▪
Inhaled anesthetics suppress excitatory synaptic transmission presynaptically by reducing glutamate release (volatile anesthetics) and postsynaptically by inhibiting excitatory ionotropic receptors activated by glutamate (gaseous and to some extent volatile anesthetics).
- ▪
Inhaled anesthetics directly activate certain two-pore-domain potassium channels, which is likely to result in both pre- and post-synaptic effects.
- ▪
There is as yet no comprehensive theory of anesthesia that describes the sequence of events leading from the interaction between an anesthetic molecule and its targets to the behavioral effects.
Despite the widespread clinical use of general anesthetics, our current understanding of their molecular, cellular, and network mechanisms is incomplete. This critical gap in the pharmacology of one of medicine’s most important drug classes not only impedes rational use of available anesthetics but also hinders the development of newer anesthetics that might selectively achieve the desirable end points of anesthesia with fewer adverse cardiovascular, respiratory, and possibly neuropathologic side effects. Although major progress has been made in understanding the pharmacology of the intravenous anesthetics by molecular genetic approaches, the actions of the inhaled anesthetics at the molecular and cellular levels are more enigmatic. It is still not possible to trace precisely the sequence of events that leads from inhaled anesthetic-target interactions, through ascending levels of biologic complexity, to the various behavioral effects that characterize the composite state of clinical anesthesia in humans. Nevertheless, investigations continue to reveal fundamental principles of action and have led to a framework for understanding anesthetic effects at different organizational levels.
The focus of this chapter is on the mechanisms involved in the principal therapeutic effects (anesthesia) and on the side effects of the inhaled anesthetics ( Fig. 19.1 ), a chemically and pharmacologically diverse group that includes the potent halogenated ether (isoflurane, sevoflurane, desflurane, enflurane) and alkane (halothane) volatile anesthetics and the inorganic gaseous anesthetics (nitrous oxide and xenon). This critical summary of the current state of knowledge begins with an historical overview and a review of the behavioral end points of anesthesia. We then trace, where possible, inhaled anesthetic effects through ascending levels of organization from molecules, cells, circuits, networks, and organs to mammalian behavior. We also briefly address studies of anesthetic effects in very simple model organisms, with anesthetic end points being identified that as yet bear uncertain relationships to those in mammals.

History
The Unified Paradigm of Anesthetic Theories
The first monograph reporting experimental work on anesthetic mechanisms, proposing a soon-to-be discredited lipid-elution theory of anesthetic action, was published only 6 months after Morton’s public demonstration of ether anesthesia in Boston’s Ether Dome. For decades thereafter, the phenomenon of anesthesia puzzled, inspired, and awed those who tried to understand it. An influential paradigm of anesthetic action formulated by Claude Bernard in the 1870s posited that anesthesia was a “unified” phenomenon―a unitary mechanism applicable to all forms of life. Although the anesthetized state could be brought about by a variety of agents, its essence was the same in all living creatures. In fact, Bernard thought that life itself was defined by susceptibility to anesthesia. Bernard also proposed a more specific theory of anesthesia, coagulation of protoplasm, which competed with a number of coexisting theories entertained by the scientific community. In a major work published in 1919, Hans Winterstein summarized the perplexing diversity of anesthetic theories by listing more than 600 references, the majority to original laboratory work—a convincing testimony to the interest of the scientific world in this phenomenon. Of note, the work of Meyer and Overton at the end of the nineteenth century had only a limited effect on the trajectory of research until the 1960s. Only then was the striking simplicity of the Meyer-Overton correlation ( Fig. 19.2 A ) of anesthetic potency with solubility in olive oil interpreted by the majority of researchers to indicate that lipids are likely to be the anesthetic target. This interpretation focused attention on anesthetic effects on the bulk physical properties of cell membranes, which were known to consist primarily of lipid molecules. Such nonspecific or “lipoid-based” anesthetic theories dominated the field from the 1960s to the 1980s.

Minimum Alveolar Concentration―A Bridge Between Past and Present
The potencies of inhaled anesthetics for immobilization were established in the classic studies in the 1960s of Eger and colleagues, who defined the minimum alveolar concentration (MAC) as the inhaled anesthetic atmospheric pressure required to prevent movement in response to a defined noxious stimulus in 50% of subjects. The concept of MAC evolved within a unitary paradigm of anesthetic action and also reflected the priorities of clinical practice. As a result, prevention of movement (immobility) became a universal yardstick for anesthetic effects, presumed to occur in the brain. Moreover, the simple elegance of the relationship between anesthetic potency and lipid solubility (see Fig. 19.2 A ) graphically illustrated Meyer and Overton’s conclusion that “All chemically indifferent substances that are soluble in fat are anesthetics. Their relative potency as anesthetics will depend on their affinity to fat on the one hand and water on the other hand, that is, on the fat/water partition coefficient.” This was interpreted as favoring lipids as the primary targets of anesthetics and a single nonspecific theory to explain anesthesia. The appeal of a single unified mechanism to explain anesthesia was (and remains) intellectually appealing. This focused the bulk of research efforts on delineating how anesthetic interactions with lipid membranes might lead to the behavioral changes observed under anesthesia―the nonspecific lipoid theory.
Because inhaled anesthetic concentrations reflect concentrations in the tissues after equilibration, which is most rapidly achieved for well-perfused organs such as the brain and heart, MAC is analogous to the plasma concentration for 50% effect (EC 50 ) for intravenous anesthetics. In clinical applications, MAC is usually expressed as volume percent (vol%), which varies considerably with temperature owing to the large temperature dependence of partitioning between the gas phase and condensed phases (be they water, lipids, or proteins), whereas the equivalent condensed-phase molar concentrations are much less dependent on temperature. The MAC concept provided researchers and clinicians with a universal standard whereby to measure a defined anesthetic end point (immobility), making meaningful comparisons of experimental results possible and accelerating clinical and laboratory research into anesthetic mechanisms. Today, a more nuanced understanding of MAC considers the structural and functional diversity of the physiologic targets for the different components of the anesthetic state.
Shift From Lipid- to Protein-Centered Mechanisms
Lipid-centered mechanisms of anesthesia prevailed in the two decades after definition of the MAC concept. Alternative targets were occasionally proposed but largely neglected by the scientific mainstream. Experimental inconsistencies of lipid targets, as well as evidence compatible with proteins as primary sites of action, were largely ignored. A shift from lipid- to protein-centered mechanisms, however, began in the late 1970s, owing largely to the discoveries of Franks and Lieb, who in an influential series of publications demonstrated that not only were lipids implausible targets but that protein targets were also compatible with the Meyer-Overton correlation (see Fig. 19.2 B )—a proof of concept that, within a few years, redirected the bulk of research efforts toward proteins. As a corollary of this reorientation, evidence against lipid-based theories was recognized. Examples include the cutoff in anesthetic potency in homologous series of long-chain anesthetic alcohols and the identification of hydrophobic drugs that do not obey the Meyer-Overton correlation. The enantiomeric selectivity of several anesthetics further strengthened the case for specific binding sites on proteins because stereoselectivity is difficult to reconcile with lipid targets. Today, there is widespread acceptance of the notion that lipid bilayers remain essentially unaffected by general anesthetics and that critical signaling proteins (e.g., ion channels or ligand-gated receptors) are the relevant molecular targets of anesthetic action. The exact identity of proteins contributing to specific anesthetic end points continues to be sought, with research addressing not only the “where” (target) but also the “how” (process) of anesthetic mechanisms.
Diversity of Potential Anesthetic Targets
At high concentrations in vitro, most inhaled anesthetics affect the functions of multiple proteins, several of which might be plausibly connected to the components of the anesthetic state or anesthetic side effects. However, when a specific anesthetic end point is considered, anesthetics are effective in vivo over a very narrow concentration range. This makes the concentration at which a relevant anesthetic effect is observed a critical consideration for deciding potential relevance. The mechanistic relevance of small effects observed in vitro at relevant concentrations is less clear; that is, what effect is too small to be considered relevant to anesthesia?
Whether anesthesia results from the sum of minor perturbations at multiple sites or from substantial effects on a small number of targets remains to be determined. This should be resolved as more sophisticated molecular genetics experimental techniques are applied to test the relevance of putative targets. There are two reasons for believing that the number of relevant targets may be small. First, the extreme steepness of anesthetic concentration-response curves means that, for a given end point, substantial effects on two or three targets would be sufficient to account entirely for the in vivo effect. Second, the stereoselectivity observed in vivo is comparable to the largest effects seen in putative targets in vitro, suggesting that only a small number of targets are likely to be involved. Set against this logic is the experimental evidence that a rather large number of plausible target proteins are affected, albeit usually to small extents, and determining which of these are relevant to the various anesthetic end points remains a challenge.
Anesthesia: A Composite Neuropharmacologic State
Along with progress in identifying the molecular mechanisms of anesthesia, our understanding of the nature of the anesthetic state has evolved. Whereas a drug-induced coma-like state of general anesthesia can be induced by inhaled anesthetics administered at appropriate concentrations (approximately 1.3 times MAC, equivalent to the EC 95 of an intravenous anesthetic), the use of such high concentrations can lead to short- and possibly long-term side effects. It is now clear that anesthesia consists of separable and at least partially independent components or substates, each of which involves distinct but possibly overlapping mechanisms in different regions of the central nervous system (CNS) and with variations in relative potencies between specific agents. Immobilization, the core measure of MAC, is mediated largely at the level of the spinal cord by inhaled anesthetics but not by barbiturates. On the other hand, the spinal cord is clearly not the primary site of such phenomena as amnesia, sedation, and unconsciousness, which rather are produced by anesthetic effects on cerebral cortical function ( Fig. 19.3 ). A functional separation between amnesia and sedation has been demonstrated for intravenous anesthetics, and it seems likely that this will apply to inhaled anesthetics as well. The state commonly referred to as “unconsciousness” is in itself heterogeneous, with evidence for distinct states of unresponsiveness and unconsciousness. These and similar findings have led to the concept that general anesthesia consists of multiple independent components that can be resolved experimentally and clinically.

In principle, each component of anesthesia can be preferentially induced in a concentration- and agent-specific manner using individual cellular/molecular pathways in various regions of the CNS. For example, injections of pentobarbital into discrete sites in the mesopontine tegmentum induce a comatose state, whereas sedation induced by systemic administration of propofol can be reversed by microinjections of γ-aminobutyric acid (GABA) A receptor antagonists into the tuberomammillary nucleus, a sleep-regulating nucleus in the hypothalamus. Thus general anesthetics produce separate identifiable anesthetic substates via agent-specific actions at discrete anatomic sites in the CNS through different molecular targets. An important consequence of this complexity is that MAC, which is based exclusively on a motor response, might not proportionately reflect other components of anesthesia. Although this heterogeneity of anesthetic actions complicates a mechanistic understanding, it does open the possibility of developing substate-specific drugs.
Integrated Effects on Central Nervous System Function
Immobility
Electroencephalography as a monitor of brain activity has been applied both to the study of anesthetic mechanisms and as a monitor of the anesthetic state. Failure to find a correlation between quantitative electroencephalographic activity and immobility in response to noxious stimulation led to the somewhat radical (at the time) hypothesis that immobility was not a cerebral cortex–mediated phenomenon. Experimental demonstration that volatile anesthetics act on the spinal cord to suppress movement supported this hypothesis and was a major factor leading to the contemporary separation of anesthetic substates, of which immobility requires the highest drug concentrations (see Fig. 19.3 ). Taking advantage of the atypical blood supply of goat CNS, which allows separate experimental perfusion of the brain and spinal cord, Antognini and colleagues showed that immobility involves anesthetic effects at the spinal level because selective delivery of isoflurane or halothane only to the brain required 2.5-fold to 4-fold higher concentrations compared with delivery to the whole CNS. At the same time, experiments by Rampil and colleagues that used surgical separation of the forebrain and midbrain from the spinal cord in rats led to the conclusion that immobilization involves primarily suppression of the nocifensive withdrawal reflex arc at the level of the spinal cord ( Fig. 19.4 ).

In the 25 years since the identification of the spinal cord as the site of anesthetic-induced immobility, research has centered on pharmacologic, genetic, and complex network approaches. The conventional pharmacologic approach (“bulk” administration of agonists and antagonists into the CNS) to identify receptor-level contributions to isoflurane-induced immobility (isoflurane being the standard potent ether for experimental purposes) has severe limitations in the complex networks of the CNS. Nevertheless, it yielded at least one surprising insight―that actions at GABA A receptors appear not to be important for the end point of immobility, at least where inhalational agents are concerned. Anesthetic-resistant transgenic mice confirmed that GABA A receptors containing α1- or α3-subunits do not contribute to the immobilizing action of isoflurane. Perhaps less surprisingly, inhibition of central nicotinic acetylcholine receptors also plays no role in immobilization. A role for voltage-gated sodium (Na + ) channels was suggested by the finding that intrathecal administration of a selective inhibitor of Na + channels potentiates anesthetic immobility (reduces MAC), whereas an enhancer of Na + channel activity does the opposite.
In contrast, work in mutant mice suggests a potential role for tandem-pore domain potassium channels (K 2P ) in anesthetic-induced immobility. Global knockout mice lacking the TASK-1, TASK-3, and TREK-1 K 2P channels are less sensitive to volatile but not intravenous anesthetics, indicating a role for these channels possibly by a presynaptic mechanism. An important limitation is that global knockout results almost invariably in wide-ranging compensatory changes in the molecular landscape of the organism with unpredictable consequences for the phenomenon under investigation.
Work with ex vivo preparations that attempt to preserve parts of the complex spinal cord circuitry suggests that anesthetic inhibition of afferent (noxious sensory) input to the dorsal horn plays a subordinate role to the suppression of the efferent (motor) output from the ventral horn, although this may vary by specific agent. This motor output is coordinated by neuronal networks organized in so-called central pattern generators that control the activity of cholinergic motoneurons. Not unlike understanding the anesthetic effects on higher cognitive function, the key to understanding immobility will likely lie in resolving the effect of anesthetics on integrated spinal network activity after understanding the circuit physiology.
Unconsciousness
Compared with the other end points of anesthesia, research into the biologic basis of anesthetic-induced unconsciousness is relatively recent, but it has become an area of active investigation. Research is being conducted in animal models and human subjects and commercial interests are working to develop effective depth-of-anesthesia monitors. These efforts reflect increasing interest, and progress, in “consciousness science” in general. Moreover, anesthetics are themselves being used as research tools to help unravel the neuronal underpinnings of consciousness.
Loss of consciousness (or hypnosis) is a hallmark of the onset of anesthesia. However, what is commonly referred to as unconsciousness under anesthesia might be more accurately described as unresponsiveness, a condition that could also encompass states of self-awareness without environmental awareness (as in dreaming) or environmental awareness without recall (e.g. conscious amnesia combined with neuromuscular paralysis during induction of anesthesia).
Numerous theories have been advanced to explain anesthetic-induced unconsciousness. They can generally be divided into those that address “bottom-up” changes in the brain stem circuitry that controls arousal, versus “top-down” changes in the thalamocortical circuits that integrate information. Indeed, this distinction formed the basis for a recent suggestion that the level of consciousness reflects bottom-up processes whereas the content of consciousness reflects top-down processes―a notion with intuitive appeal.
One of the most influential theories has been the “integrated information theory of consciousness (IITC)” of Tononi, which emphasizes the need for simultaneous differentiation between brain states and their integration into a coherent whole. Drugs or diseases that suppress consciousness could act through either process. Other information-based approaches use symbolic analysis, transfer entropy, chaos theory, and more. The rich connectivity of the cerebral cortex and its hierarchical organization are especially suited to enable high levels of information integration in the human brain. Some brain areas present a “rich club” organization (i.e., highly connected nodes tend to be preferentially connected to other highly connected nodes), which has been suggested to be optimal for information integration. These hubs are promising targets for the hypnotic action of general anesthetic drugs.
Anesthetics might act by interfering with the operational synchronicity and coherence of corticothalamic networks. Consequent disruption of functional and effective connectivity has been observed during natural slow-wave and midazolam-induced loss of responsiveness. This breakdown of cortical connectivity, rather than pharmacologic deafferentation from the environment, could underlie loss of consciousness. Unconsciousness would then be characterized not by the absence but by the fragmentation of cortical processing.
Although the mechanism of “binding” (i.e., creating the unity of perception) is uncertain, synchronicity of neuronal activity in the 40- to 90-Hz range across functionally connected cortical areas (commonly referred to as 40-Hz- or γ-rhythm) is a viable candidate. Animal and human data implicate activity in the γ-band throughout the cortex as a network-level target of general anesthetics. Anesthetic actions on cortical information processing probably consist not merely of suppression of responses but of reduced complexity and variability reflected counterintuitively in the increased reliability and precision of evoked responses.
A consistent and intriguing observation has been that anesthetics suppress descending more than ascending neural connectivity. Within the framework of predictive coding, this indicates that unconsciousness is associated with a reduction in internally generated predictions more than a suppression of incoming sensory information. The molecular and cellular mechanisms underlying this effect remain undefined, but the preferential suppression by isoflurane of cortico-cortical responses in brain slices in vitro supports a top-down mechanism wherein the anesthetic acts directly on the thalamocortical circuitry.
By contrast, “bottom-up” theories attribute changes in consciousness to anesthetic modulation of subcortical arousal nuclei. An interesting theme has emerged from this line of research. There is substantial overlap between the centers whose activity is altered during natural slow-wave sleep and the state of general anesthesia. That is, many anesthetics may induce unconsciousness, at least in part, by “hijacking” neural sleep or arousal pathways.
Thalamic theories of anesthetic-induced unconsciousness incorporate aspects of both top-down and bottom-up mechanisms, reflecting not only the intermediate position of this structure in the hierarchical organization of the brain but also the different connection patterns of “sensory relay” versus higher-order “nonspecific” thalamic nuclei.
Learning and Memory
Anterograde amnesia, one of the core desirable anesthetic end points, is achieved at lower anesthetic concentrations (∼0.25 MAC) than those required for unconsciousness (∼0.5 MAC). Perhaps the closest analogue in rodents to explicit memory in humans is medial temporal lobe–dependentlearning of temporal and spatial sequences known as hippocampus-dependent spatial learning . Other learning paradigms, such as fear conditioning to tone, are by contrast independent of the hippocampus. Spatial learning can be tested by a variety of experimental paradigms, including fear conditioning to context ( Fig. 19.5 ). Isoflurane and the nonimmobilizer F6 both inhibit hippocampus-dependent learning at about half the concentration necessary for disrupting hippocampus-independent learning. Similarly, anesthetic concentrations that inhibit explicit memory in humans (memory that can be explicitly recalled as opposed to motor learning, classical conditioning, and so on) are similarly lower than concentrations that impair implicit memory (not subject to willful recollection). Taken together, these findings implicate effects on function of the medial temporal lobe, including the hippocampus, in the suppression of explicit memory by anesthetics. Effects on other structures, such as the amygdala, may be relevant to anesthetic impairment of implicit or other types of memory.

Because inhaled anesthetics affect multiple cellular targets even at amnesic concentrations, it is likely that anesthetic-induced amnesia arises from multiple cellular-level changes. A quantitative comparison of the degree of change in synaptic inhibition in the hippocampus produced by equiamnestic concentrations of isoflurane versus etomidate indicated that the enhancement of GABAergic inhibition can account for a substantial portion of isoflurane’s effect on memory. Other contributing targets may include nAChRs, HCN1 channels, and excitatory glutamatergic synapses, Conversely, it is also likely that suppression of learning and memory by drugs known to have different receptor affinities share common mechanisms at some level of integration. For example, θ-rhythms (4-12 Hz) are clearly important for hippocampus-dependent learning and memory. Benzodiazepines and cannabinoids slow and suppress hippocampal θ-rhythms in proportion to their ability to impair hippocampus-dependent learning. Isoflurane and the nonimmobilizer F6 have comparable effects on θ-rhythms at amnesic concentrations while having different receptor-level profiles and opposite effects on sedation. Thus alterations in neuronal synchrony may provide a common network-level substrate for memory impairment. The synchronization between amygdalar and hippocampal θ-rhythms that occurs during fear memory retrieval indicates that this principle might also apply to other forms of memory and their impairment by anesthetics. As with other components of the anesthetic state, the precise mechanisms of memory impairment by anesthetics and of memory itself remain to be fully elucidated.
Sedation
Sedation (defined as a decrease in activity, alertness, arousal, and/or vigilance), which is on a behavioral continuum leading to hypnosis, is achieved at anesthetic doses similar to those that produce amnesia (<0.5 MAC). There is no clear mechanistic or clinical separation between sedation and hypnosis. By contrast, even though sedation can be difficult to separate from amnesia, for intravenous anesthetics there may be separate but overlapping substrates for these two end points. The mechanisms involved in these behavioral effects are likely to resemble those of less promiscuous drugs, for which genetic approaches have been informative. An amino acid knockin mutation (H101R) in mice that renders the α 1 GABA A receptor subunit insensitive to modulation by benzodiazepines produces resistance to the sedative and amnesic effects of benzodiazepines while maintaining other behavioral effects, among them anxiolysis. The α 1 subunit is abundantly expressed in the CNS, mainly in the cortical areas and thalamus. Volatile anesthetics have qualitatively similar effects on α 1 -containing GABA A receptors (but also those containing other subunits) at low concentrations. The observation that the nonimmobilizer F6, which is devoid of sedative properties, is amnesic but does not modulate benzodiazepine-sensitive α 1 -containing GABA A receptors, is compatible with a role for α 1 -containing receptors in volatile anesthetic-induced sedation, because few other targets are affected at purely sedative concentrations. Possible targets for the sedative effects of the gaseous anesthetics nitrous oxide and xenon, which do not affect GABA A receptors, include N -methyl-d-aspartate (NMDA) receptor antagonism and K 2P channel activation. Consistent with this distinct pharmacologic profile, nitrous oxide has strikingly different effects from those of benzodiazepines in behavioral tests aimed at evaluating sedation in mice.
Recognizing that there may be more than a superficial similarity between natural sleep and anesthetic-induced sedation and hypnosis, the effects of some anesthetics apparently share natural sleep mechanisms by directly activating discrete sleep-promoting nuclei in the hypothalamus. Indeed, the same neurons, or at least overlapping populations of neurons, that are activated by sleep deprivation are also activated during dexmedetomidine-induced sedation. Electroencephalographic patterns during natural slow-wave sleep and anesthesia show similarities, and recovery from sleep deprivation can occur under propofol and inhalational anesthesia, supporting this concept. Anesthetic effects on other cortical and subcortical structures may also contribute to anesthetic-induced sedation and hypnosis.
Identification of Molecular Sites of Anesthetic Action
Criteria for Identifying Sites Relevant to Anesthesia
Specific criteria have been proposed to evaluate the relevance of the many potential molecular targets of anesthetics. These criteria include the following:
- 1.
Reversible alteration of target function at clinically relevant concentrations. This criterion requires comparable in vivo and in vitro sensitivities and depends on the anesthetic end point under consideration. For example, targets involved in immobility must be sensitive to anesthetics near MAC, whereas targets mediating amnesia must be affected at a fraction of MAC. Recent evidence for persistent effects of inhaled anesthetics demonstrable in the absence of continued anesthetic exposure is challenging the notion of reversibility for certain effects.
- 2.
Expression of the target in appropriate anatomic locations to mediate the specific anesthetic end point. For example, immobilization by inhaled agents appears to involve primarily actions in the spinal cord independent of actions in the brain.
- 3.
Concordant stereoselectivity of anesthetic effects in vivo and on the target in vitro. Without a specific pharmacologic antagonist of anesthesia, correlation between the stereoselective actions of general anesthetics in vivo and in vitro is a useful test of pharmacologic relevance of putative molecular targets. Stereoselectivity data correlating in vivo potency and in vitro receptor actions implicate GABA A receptors as a target for the anesthetic actions of etomidate, pentobarbital, neurosteroid anesthetics, and isoflurane.
- 4.
Appropriate sensitivity or insensitivity to anesthetic and nonanesthetic compounds. Anesthetic halogenated cyclobutanes together with structural analogs that do not produce anesthesia at concentrations predicted to be anesthetic by the Meyer-Overton correlation (nonimmobilizers) can be used to discriminate relevant volatile anesthetic targets in vitro. For example, the anesthetic F3 (1-chloro-1,2,2-trifluorocyclobutane), but not the structurally similar nonanesthetic F6 (1,2-dichlorohexa fluorocyclobutane), affect GABA A , glycine, AMPA (α-amino-3-hydroxy-5-methyl-4-isoxazolepropionic acid), kainate, and 5-HT3 receptors; and Na + channels, consistent with possible roles in immobility, whereas both F3 and F6 affect neuronal nicotinic, M1 muscarinic, 5-HT2C, and mGluR5 receptors, indicating that these targets are not involved in immobility. F6 is interesting in that it lacks sedative and immobilizing effects but does possess amnesic effects, hence use of the more accurate term nonimmobilizer , making it a useful pharmacologic tool for discriminating targets for these actions.
- 5.
Predictable effects of genetic manipulations targeted to putative molecular targets. The effects of targeted deletion of specific molecules implicated as anesthetic targets (knockout mutations) or genetic engineering to introduce specific mutations that modify anesthetic sensitivity (knockin mutations) in model organisms provide powerful approaches to test the roles of putative molecular targets of anesthetic action. This approach has been particularly successful in implicating specific GABA A receptor subtypes in the effects of the GABAergic intravenous anesthetics propofol and etomidate, where single amino acid substitutions in specific receptor subtypes eliminate anesthetic effects both in vitro and in vivo. Targeted mutations of putative anesthetic targets provide a bridge between in vitro observations and whole-animal experiments essential for demonstrating anesthetic end points. The existence of multiple targets and redundancy among ion channel subtypes makes this a more challenging experimental approach for inhaled anesthetics compared with intravenous anesthetics (discussed later).
Physicochemical Properties of Anesthetic Binding Sites
A convergence of x-ray crystallography, molecular modeling, and structure-function studies indicates that inhaled anesthetics bind in the hydrophobic cavities formed within proteins. The lipophilic (or hydrophobic) nature of these binding sites explains their adherence to the Meyer-Overton correlation. An element of amphiphilicity (possessing both polar and nonpolar characteristics) is also required for effective interaction with these cavities, as indicated by improvements in the Meyer-Overton correlation with more amphipathic solvents (possessing both hydrophobic and hydrophilic properties).
From Model Proteins to Receptors
Identifying inhaled anesthetic binding sites on plausible target proteins is difficult because of their low-affinity interactions, the paucity of atomic resolution structures of pharmacologically relevant target proteins, and the lack of specific antagonists. Consequently most anesthetic binding sites have been identified in well-characterized model proteins for which three-dimensional atomic resolution structures―such as luciferase and serum albumin ―are available but are not themselves relevant to anesthesia. These studies indicate that anesthetics bind in pockets with both nonpolar and polar noncovalent chemical interactions. Binding involves weak hydrogen bond interactions with polar amino acid residues and water molecules, nonpolar van der Waals interactions, and a polarizing effect of the amphiphilic binding cavity on the relatively hydrophobic anesthetic molecules. Occupation of a site, or sites, by an anesthetic provides a plausible mechanism for alteration of receptor and ion channel function by selectively binding to a particular conformation (e.g., an open or inactivated state of an ion channel). Studies of glycine, GABA A , and NMDA receptors provide convincing evidence for the existence of anesthetic binding sites in critical neuronal signaling proteins. It is likely that before long, high-resolution crystal structures of these receptors will be determined with inhaled anesthetic bound. However, because anesthetics act by binding only to certain transient conformational states, the relevance of necessarily static crystal structures will have to be assessed with care.
Structural studies using the more accessible prokaryotic homologues of eukaryotic ion channels have provided a powerful tool for the study of anesthetic binding sites in biologically plausible proteins. For example, both propofol and desflurane have been cocrystalized with Gloebacter violaceus , a bacterial homologue of eukaryotic inhibitory ligand-gated ion channels (glycine and GABA A receptors). Both anesthetics bind to a common preexisting site in the upper part of the transmembrane domain between the transmembrane segments of a single subunit ( Fig. 19.6 ). Molecular modeling based on structurally homologous proteins has also been used to identify putative anesthetic binding sites in the transmembrane domains of vertebrate GABA A and glycine receptors ( Fig. 19.7 ). These models suggest that different drugs may either bind in different orientations within a single amphiphilic cavity or occupy different cavities within the protein, causing similar functional effects. Refinement of these molecular models will continue to provide new insights in the molecular basis for general anesthetic action that can be experimentally tested. For example, potential sites of interaction of xenon and isoflurane with the NMDA receptor have also been identified using this approach. One site, which can contain up to three xenon atoms or one molecule of isoflurane, overlaps the known binding site for the coagonist glycine in the NR1 subunit. This suggests that two chemically dissimilar inhaled anesthetics inhibit NMDA receptors by direct competitive inhibition of coagonist binding.


Molecular Targets of Inhaled Anesthetics
Ion channels have emerged as the most promising molecular targets for inhaled anesthetics. Neurotransmitter-gated ion channels―in particular GABA A , glycine, and NMDA-type glutamate receptors―are leading candidates owing to their appropriate CNS distributions, essential physiologic roles in inhibitory and excitatory synaptic transmission, and sensitivities to clinically relevant concentrations of anesthetics. Other ion channels that are sensitive to inhaled anesthetics include the hyperpolarization-activated cyclic nucleotide (HCN)-gated family of channels that give rise to pacemaker currents and regulate dendritic excitability, two-pore domain (K 2P ) “leak” K + channels that maintain resting membrane potential in many cells, and voltage-gated Na + and Ca 2+ channels.
Ligand-Gated ION Channels
Potentiation of Inhibitory GABA A and Glycine Receptors
The ether anesthetics (including isoflurane, sevoflurane, and desflurane), the alkane anesthetic halothane, most intravenous anesthetics (including propofol, etomidate, barbiturates), and the neurosteroid anesthetics enhance GABA A and glycine receptor (GlyR) function. GABA A and GlyRs are members of the same cys-loop ligand-gated ion channel superfamily that also includes the cation-permeable nicotinic acetylcholine and 5HT 3 receptors. GABA A receptors are the principal transmitter-gated Cl − channels in the neocortex and allocortex, whereas GlyRs fulfill this function in the spinal cord, with some overlap in the diencephalon and brain stem. Activated receptors conduct chloride ions, driving the membrane potential toward the Cl − equilibrium potential. Both receptors are inhibitory (except in some cases during development) because the Cl − equilibrium potential is usually more negative than the normal resting potential. Channel opening also reduces membrane resistance and “shunts” excitatory responses. Most functional GABA A and GlyRs are heteropentamers, typically consisting of three different GABA A subunits (e.g., two α, two β, and one γ or ∂) or two different GlyR subunits (three α and two β). The subunit composition of GABA A receptors determines their physiologic and pharmacologic properties and varies between and within brain areas as well as between different compartments of individual neurons. Examples are the preferential expression of the α 5 subunit in the dendritic field of the hippocampal CA1 area (a region important for memory formation), of the α 4 subunit in the thalamus, and of the α 6 subunit in the cerebellum. Presence of a γ subunit is required for benzodiazepine modulation of GABA A receptors and can also influence modulation by inhaled anesthetics. Although the molecular mechanisms of receptor modulation by inhaled anesthetics are not clear, these receptors have been key to our understanding of anesthetic-receptor interactions. Using chimeric receptor constructs between anesthetic-sensitive GABA A and insensitive GlyR subunits, specific amino acid residues in transmembrane domains 2 and 3 critical to the action of inhaled anesthetics have been identified. This laid the groundwork for the construction of anesthetic-resistant GABA A receptors and the generation of transgenic mice with altered anesthetic sensitivity (discussed later).
The related cation-permeable 5-hydroxytryptamine (serotonin)-3 (5HT 3 ) receptors are similarly potentiated by volatile anesthetics. 5HT 3 receptors are involved with autonomic reflexes and also probably contribute to the emetogenic properties of volatile anesthetics.
Inhibition of Excitatory Acetylcholine and Glutamate Receptors
Neuronal nicotinic acetylcholine receptors (nnAChRs), like the other members of the cys-loop family, are heteropentameric ligand-gated ion channels but are cation-selective. They are composed of α and β subunits but functional homomeric receptors can be formed by certain α subunits. In the CNS, nnAChRs are localized primarily presynaptically. Homomeric α 7 receptors have high permeability to Ca 2+ , which can exceed that of NMDA receptors. In contrast to GABA A and GlyRs, nnAChRs pass cations when activated and therefore depolarize the membrane potential. Receptors containing α 4 β 2 subunits are very sensitive to block by isoflurane. Relevance of nnAChR block to immobilization, sedation, and unconsciousness by inhaled anesthetics is unlikely because nnAChRs are also blocked by nonimmobilizers, although it is possible that they do contribute to amnesia.
NMDA receptors are a major postsynaptic receptor subtype of inotropic receptors for glutamate, the principal excitatory neurotransmitter in the mammalian CNS. Typical NMDA receptors, defined pharmacologically by their selective activation by the exogenous agonist NMDA, are heteromers consisting of an obligatory GluN1 subunit and modulatory GluN2 subunits. Channel opening requires glutamate (or another synthetic agonist such as NMDA) binding to the GluN2 subunit while the endogenous coagonist glycine binds to the GluN1 subunit. NMDA receptors also require membrane depolarization to relieve voltage-dependent block by Mg 2+ . Depolarization is typically provided by the binding of glutamate to non-NMDA glutamate receptors (discussed later). Because of this requirement for both presynaptic transmitter release and postsynaptic depolarization, synaptic NMDA receptors function as coincidence detectors, and this characteristic is thought to be central to their role in learning and memory. NMDA receptors are also involved in the development of chronic pain, perhaps because of mechanisms similar to those underlying synaptic plasticity, and in ischemia-induced excitotoxicity by virtue of their capacity to allow entry of the ubiquitous intracellular signal Ca 2+ . The nonhalogenated inhaled anesthetics xenon, nitrous oxide, and cyclopropane have minimal effects on GABA A receptors but depress excitatory glutamatergic synaptic transmission postsynaptically via NMDA glutamate receptor blockade ( Fig. 19.8 ). Volatile anesthetics can also inhibit isolated NMDA receptors at higher concentrations. Along with presynaptic inhibition of glutamate release, this might contribute to their depression of NMDA receptor–mediated excitatory transmission.

A second class of inotropic glutamate receptors includes the non-NMDA receptors, which are subdivided into AMPA and kainate receptors based again on their sensitivities to selective exogenous agonists. Inhaled anesthetics only weakly inhibit AMPA receptors; hence this action is unlikely to be important in their actions. Interestingly, kainate receptors are enhanced by inhaled anesthetics, but this is unlikely to be involved in immobility because MAC is not altered in mice deficient in the GluR6 receptor subunit. Most evidence suggests that the principal mechanism for depression of glutamatergic transmission by volatile anesthetics is presynaptic, with minor contributions from postsynaptic receptor blockade (see section on “Cellular Mechanisms”).
Voltage-Gated and other ION Channels
Volatile anesthetics have diverse effects on a wide variety of ion channels that are critically involved in their neurophysiologic, cardiovascular, and respiratory actions. A detailed understanding of these interactions is crucial to a pharmacologic description of this essential drug class.
Na + Channels
Voltage-gated Na + channels are critical to axonal conduction, synaptic integration, and neuronal excitability. In contrast to findings in invertebrate giant axons, axonal conduction in small (0.1-0.2 μm) unmyelinated hippocampal axons is depressed by volatile anesthetics, and small reductions in preterminal action potential amplitude significantly depress transmitter release and hence postsynaptic responses at a mammalian synapse. Heterologously expressed mammalian voltage-gated Na + channels are sensitive to clinically relevant concentrations of volatile anesthetics. The Na + channel family consists of nine homologous pore-forming α subunits with distinct cellular and subcellular distributions. Isoflurane and other volatile anesthetics inhibit the major mammalian Na + channel isoforms, including neuronal (Na v 1.2), skeletal muscle (Na v 1.4), cardiac (Na v 1.5), and peripheral (Na v 1.8) isoforms. Volatile anesthetics but not nonimmobilizers also inhibit native neuronal and nerve terminal Na + channels, lending support to the notion that Na + channel blockade contributes to the depression of synaptic neurotransmitter release. In contrast, xenon has no detectable effect on Na + , Ca 2+ , or K + channels in isolated cardiomyocytes. The demonstration that NaChBac, a prokaryotic homologue of voltage-gated Na + channels, is also inhibited by volatile anesthetics opens the way for structure-function studies of these channels. These studies suggest that volatile anesthetics affect channel gating, probably via at least two drug binding sites.
Ca 2+ Channels
Multiple cellular functions depend on the tightly controlled concentration of intracellular free Ca 2+ ([Ca 2+ ] i ), which is determined by the integrated activities of voltage-gated Ca 2+ channels, capacitative Ca 2+ channels, plasma membrane and sarcoplasmic/endoplasmic reticulum (ER) Ca 2+ -adenosine triphosphatases (pumps), Na + /Ca 2+ exchangers, mitochondrial Ca 2+ sequestration, and cytoplasmic Ca 2+ -binding proteins. Alteration of any of these mechanisms can affect the many cellular processes regulated by the second-messenger actions of Ca 2+ , including synaptic transmission, gene expression, cytotoxicity, and muscle excitation-contraction coupling. Excitable cells translate their electrical activity into action by Ca 2+ fluxes mediated primarily by voltage-gated Ca 2+ channels in the plasma membrane. Distinct Ca 2+ channel subtypes are expressed in various cells and tissues, and are classified pharmacologically and functionally by the degree of depolarization required to gate the channel as low voltage–activated (LVA; T-type) or high voltage–activated (HVA; L-, N-, R-, and P/Q-type) channels. Cloning and sequencing to identify their pore-forming α subunits has allowed molecular classification of these functionally identified channel subtypes. Considerable evidence indicates that volatile anesthetics inhibit certain Ca 2+ channel isoforms but not others.
Inhibition of presynaptic voltage-gated Ca 2+ channels coupled to transmitter release has been proposed as a mechanism by which volatile anesthetics reduce excitatory transmission. Indeed, N-type (Ca v 2.2) and P-type (Ca v 2.1) channels, which mediate Ca 2+ entry coupled to neurotransmitter release, are modestly sensitive to volatile anesthetics but not in all neuron types, suggesting the importance of auxiliary subunits, posttranslational modification, or other potential modulators of anesthetic sensitivity. A modest contribution of R-type Ca 2+ channels (Ca v 2.3) to anesthesia is suggested by their sensitivity to volatile anesthetics and a small increase in MAC produced by genetic deletion in mice. T-type Ca 2+ channels are particularly sensitive to volatile anesthetics and nitrous oxide. However, mutant mice lacking a major neuronal T-type Ca 2+ channel isoform (Ca v 3.1) have normal volatile anesthetic sensitivity, although the onset of anesthesia is delayed. Thus the role that inhibition of these or other Ca 2+ channels plays in the CNS effects of inhaled anesthetics is unclear.
At higher doses, a role for Ca 2+ channel inhibition in the negative inotropic effects of volatile anesthetics is well established. The force of myocardial contraction is determined by the magnitude of cytosolic Ca 2+ increase after electrical excitation, the responsiveness of the contractile proteins to Ca 2+ , and sarcomere length. Negative inotropic effects of volatile anesthetics are mediated by reductions in Ca 2+ availability, Ca 2+ sensitivity of the contractile proteins, and rate of cytosolic Ca 2+ clearance. Volatile anesthetics reduce the Ca 2+ transient and shorten action potential duration in cardiomyocytes primarily by inhibiting L-type (Ca v 1.2) Ca 2+ currents, resulting in a negative inotropic effect and arrhythmogenicity. In contrast, xenon does not depress myocardial function or inhibit L-type Ca 2+ , Na + , or K + currents in isolated cardiomyocytes. Inhibition of trans-sarcolemmal Ca 2+ influx through cardiac L-type Ca 2+ channels plays a major role in the negative inotropic effects of volatile anesthetics—greatest for halothane—along with contributions from effects on myofilament Ca 2+ sensitivity and sarcolemmal Ca 2+ release.
In contrast to voltage-gated Ca 2+ channels that regulate the influx of extracellular Ca 2+ , intracellular Ca 2+ channels regulate Ca 2+ release from intracellular stores, particularly the ER and sarcoplasmic reticulum (SR). These include 1,4,5-inositol triphosphate receptors (IP 3 Rs), regulated by the second messenger IP 3 , and ryanodine receptors (RyRs); the latter mediate the release of SR Ca 2+ , which is critical to excitation-contraction coupling in muscle. Volatile anesthetic–induced Ca 2+ leak occurs by effects on both IP 3 R and RyR channels, which leads to depletion of intracellular Ca 2+ stores from the SR and ER. In brain, volatile anesthetic activation of IP 3 Rs has been proposed as a mechanism for anesthetic neurotoxicity. This blunts changes in intracellular Ca 2+ in response to stimulation and contributes to the smooth muscle–relaxing properties of volatile anesthetics that underlie bronchodilation and vasodilation. Malignant hyperthermia is a pharmacogenetic disorder that manifests as a potentially fatal hypermetabolic crisis triggered by volatile anesthetics, particularly halothane. It is often associated with mutations in RyR1 and the physically associated L-type Ca 2+ channel (Ca v 1.1), which functions as the voltage sensor. Volatile anesthetics activate the mutated RyRs, resulting in uncontrolled intracellular Ca 2+ release from the SR, muscle contraction, and hypermetabolic activity.
K + , HCN, and TRP Channels
Potassium (K + ) channels are members of an extremely diverse ion channel family noted for their varied modes of activation. They regulate electrical excitability, muscle contractility, and neurotransmitter release. They are important in determining input resistance and in driving repolarization after action potentials; thus they determine excitability and action potential duration. Given the large diversity in K + channel structure, function, and anesthetic sensitivity, it is not surprising that there is considerable diversity in their sensitivity and response to inhaled anesthetics, from relatively insensitive (voltage-gated K + channels K v 1.1, K v 3) to sensitive (some members of the K 2P family), resulting in either inhibition, activation, or no effect on K + currents.
Volatile anesthetic activation of certain “leak” K + channels was first observed in the snail Lymnaea , although the molecular identity of the affected ion channels was unknown. Activation of K 2P channels by volatile and gaseous anesthetics―including xenon, nitrous oxide, and cyclopropane―was subsequently observed in mammals. Increased K + conductance can hyperpolarize neurons, reducing responsiveness to excitatory synaptic input and possibly altering network synchrony. Targeted deletion of the TASK-1, TASK-3, and TREK-1 K 2P channels in mice reduces sensitivity to immobilization by volatile anesthetics in an agent-specific manner, implicating these channels as contributory anesthetic targets in vivo. The K + channel TREK-1 also contributes to the neuroprotective effects of xenon and sevoflurane.
Progress has recently been made in identifying anesthetic binding sites to K + channels using photoaffinity labeling, which has identified a sevoflurane binding site in the K v 1.2 channel; this is widely expressed in brain and positively modulated by volatile anesthetics. Molecular modeling has also been used to identify likely binding sites in K 2P channels.
The recognition that inherited channelopathies are arrhythmogenic and constitute an important contributor to sudden cardiac death, particularly in young children, highlights the importance of analyzing anesthetic modulation of cardiac ion channels. Recombinant hERG (human ether-a-go-go–related) channels are moderately inhibited by halothane, and their depression likely contributes to arrhythmogenic effects of volatile anesthetics ; they are also involved in acquired (drug-induced) and inherited long QT syndrome. Cardiac inward-rectifying (K IR ), voltage-gated (K v ), and Ca 2+ -activated K + channels are generally relatively insensitive to clinical concentrations of volatile anesthetics and xenon. In contrast, volatile anesthetics and xenon activate cardiac mitochondrial and sarcolemmal K ATP channels, which might contribute to anesthetic preconditioning to cardiac ischemia. Direct electrophysiologic effects of anesthetics with preconditioning properties have been demonstrated on both mitochondrial and sarcolemmal K ATP channels, although the precise mechanisms remain to be clarified. The large conductance (BK) mitochondrial K + channel Slick of the Slo2 gene family has been shown to be important for volatile anesthetic preconditioning in vivo.
Volatile anesthetics also inhibit HCN pacemaker channels, reducing the rate of rise of pacemaker potentials and the bursting frequency of certain neurons that show autorhythmicity. They decrease the I h conductance in neurons and modulate recombinant HCN1 and HCN2 channel isoforms at clinically relevant concentrations. Because HCN channels contribute to resting membrane potential, control action potential ring, dendritic integration, neuronal automaticity, and temporal summation, and also determine periodicity and synchronization of oscillations in many neuronal networks, anesthetic modulation of these channels could play an important role in anesthetic effects on neuronal integrative functions. Selective knockout of HCN1 in mouse forebrain shows a role for these channels in the amnestic and hypnotic but not immobilizing effects of volatile anesthetics.
Intracellular Signaling Mechanisms
Cell signaling mechanisms are critical to all phases of organ and cellular function, which has made them attractive targets for producing the broad effects of general anesthetics on multiple organs. Anesthetics have complex actions on intracellular cell signaling pathways, which include processes downstream from cell surface receptors and ion channels, including effects on second messengers, protein phosphorylation pathways, and other regulatory mechanisms.
G-Protein–Coupled Receptors
A variety of signals―including hormones, neurotransmitters, cytokines, pheromones, odorants, and photons―produce their intracellular actions by interactions with metabotropic receptors that activate heterotrimeric guanine nucleotide–binding proteins (G proteins). In contrast to inotropic receptors that directly couple to ion-selective channels, G proteins act as indirect molecular switches to relay information from activated plasma membrane receptors to appropriate intracellular targets.
Heterotrimeric G proteins consist of a large α-subunit and a smaller β/γ-subunit dimer, each expressed as multiple isoforms with distinct properties and downstream targets. G proteins regulate a plethora of downstream effectors to control the levels of cytosolic second messengers such as Ca 2+ , cyclic adenosine monophosphate, and inositol triphosphate. These, in turn, regulate effector proteins such as ion channels and enzymes, either directly or via second messenger–regulated protein phosphorylation pathways. Ca 2+ is a ubiquitous second messenger that regulates a number of downstream effectors, often mediated by the multifunctional Ca 2+ -binding protein calmodulin. As volatile anesthetics have profound effects on intracellular Ca 2+ concentrations via their effects on both plasma membrane and intracellular Ca 2+ channels, transporters, and exchangers (see the section on ion channels earlier), many of the downstream effects of anesthetics are ultimately mediated by changes in the second-messenger actions of Ca 2+ .
Drugs that act through G protein–coupled receptors (GPCRs) such as agonists for μ-opioid and α 2 -adrenergic receptors can affect anesthetic sensitivity (reduce MAC). Inhaled anesthetics can also directly affect signaling via GPCRs. For example, volatile anesthetics activate multiple olfactory GPCRs in the rat in vivo in a receptor- and agent-selective manner. Analogous effects on related GPCRs more relevant to critical anesthetic end points are possible but remain to be demonstrated. The observation that both volatile anesthetics and nonimmobilizers inhibit mGluR5 glutamate receptors, 5-HT 2A serotonin receptors, and muscarinic acetylcholine receptors suggests that these GPCR effects do not contribute to anesthetic immobilization.
Protein Phosphorylation
Phosphorylation of proteins on specific serine, threonine, or tyrosine hydroxyl groups, a posttranslational modification involved in the regulation of many anesthetic-sensitive receptors and ion channels, is pivotal to synaptic plasticity (e.g., long-term potentiation [LTP]). Phosphorylation is controlled by the balance of activity between protein kinases and phosphatases, several of which are plausible anesthetic targets. The protein kinase C (PKC) family of multifunctional protein kinases is activated by the lipid-signaling molecule diacylglycerol and is involved in the regulation of many ion channels and receptors. Halothane and sevoflurane enhance the activity of some PKC isoforms and stimulate phosphorylation of specific PKC substrates. Structural studies have identified a potential binding site in the diacylglycerol binding domain of PKCδ, consistent with the ability of certain anesthetics to mimic this natural regulator by binding to the activating site. A specific role for a direct pharmacologically relevant effect related to general anesthesia mediated by anesthetic activation of PKC or of any other kinase has yet to be demonstrated. Intrathecal injection of isoform-specific inhibitors of PKC does not affect sensitivity to halothane in vivo. Knockout mice lacking the PKCγ isoform show normal sensitivity to halothane and desflurane while isoflurane MAC was increased, suggesting that PKC is not critical to volatile anesthetic immobilization.
An important role for effects of volatile anesthetics and xenon on cell signaling mechanisms has been discovered for anesthetic-induced preconditioning in the heart and brain against ischemic damage. Anesthetic-induced and ischemic cardiac preconditioning share critical signaling mechanisms, including activation of multiple GPCRs (e.g., adenosine, opioid, adrenergic) and protein kinases (e.g., src kinase, PKCδ, PKCε, Akt, mitogen-activated protein kinases [MAPKs]), and their downstream targets, particularly sarcolemmal and/or mitochondrial K ATP channels, possibly initiated by changes in reactive oxygen species as the critical second messenger. Volatile anesthetics and xenon share cardioprotective and neuroprotective effects involving these signaling pathways.
The effects of anesthetics on the phosphorylation of individual residues in specific substrates can be studied using phosphorylation state–specific antibodies that are able to detect the phosphorylated forms of kinase substrates. A comparison of the effects of three mechanistically diverse anesthetics (isoflurane, propofol, and ketamine) on critical intracellular protein phosphorylation signaling pathways that are known to integrate multiple second messenger systems reveals both shared and agent-specific actions in vivo. All three anesthetics reduce phosphorylation of activating sites on NMDA and AMPA glutamate receptors and of the downstream extracellular signal-regulated kinase ERK2, all of which are known to be involved in synaptic plasticity, consistent with depression of normal glutamatergic synaptic transmission in the anesthetized mouse cerebral cortex. These effects are somewhat selective in that several other protein kinase A (PKA) substrates examined are not affected, indicating substrate-specific effects rather than a general inhibition of PKA activity. Additional studies will be required to determine which anesthetic effects on kinase pathways represent direct effects, as occurs with PKC, and which are indirect because of anesthetic-induced alterations in signaling molecules known to regulate protein kinase and phosphatase activity such as Ca 2+ and other second messengers.
Gene Expression
The ability of general anesthetics to alter gene expression in the brain was first observed for the highly reactive immediate early genes c-fos and c -jun . Anesthetic effects on gene expression have since been observed for multiple anesthetics and organs. In the hippocampus of aged rats, changes in gene expression persisted for up to 2 days in rats exposed to isoflurane and nitrous oxide, and changes in protein expression have been observed 3 days after exposure to desflurane. The significance of these changes in gene and protein expression persisting after recovery from the classic signs of anesthesia remains to be established (see review ). Recent findings suggest that certain anesthetic effects are mediated by epigenetic mechanisms involving specific posttranslational modifications of DNA-binding histone proteins by acetylase and deacetylase enzymes. For example, exposure of neonatal rats to general anesthesia led to reduced histone 3 acetylation and delayed cognitive deficits that could be reversed by a histone deacetylase inhibitor.
Cellular Mechanisms
Neuronal Excitability
Neuronal excitability is determined by resting membrane potential, threshold for action potential initiation, and input resistance (an indication of overall channel activity). Considerable diversity exists for each of these factors in different types of neurons, and membrane properties differ not only between neurons but also between compartments (e.g., soma versus dendrite). Moreover, anesthetic effects vary with the state of the individual neuron—that is, whether it is hyperpolarized or depolarized, stimulated by synaptic inputs, or quiescent. Therefore results obtained using model systems or reduced preparations (e.g. cultured neurons and brain slices) incompletely reflect anesthetic effects in vivo. Nevertheless much valuable information has been obtained using these approaches because they allow changes in neuronal activity to be attributed to specific cellular and molecular targets.
The intrinsic excitability of spinal motoneurons was reported to be little affected by halothane, but changes in hippocampal pyramidal neuron excitability were shown to be substantial and complex. Threshold can be increased or decreased, and regional differences and dose-dependent effects on firing patterns have been observed. Neurons in the ventral-posterior nucleus of the thalamus (possibly thalamic relay neurons) hyperpolarize in the presence of isoflurane and are less likely to fire action potentials because of a decrease in input resistance (increased shunting)―an effect that has been attributed to increased K + conductance. Similar effects are observed in hypoglossal motoneurons and neurons from the locus coeruleus, where a TASK-type K 2P channel has been causally implicated.
GABA A receptors located at extrasynaptic sites can also influence excitability by increasing membrane conductance and thereby “shunting” excitatory currents. These receptors have a high affinity for GABA; they are continually exposed to low ambient GABA concentrations and desensitize slowly. Their importance to anesthesia, however, may hinge on exactly what these ambient concentrations are. Tonic current in hippocampal pyramidal neurons arises primarily from α 5 subunit–containing GABA A receptors, which are highly sensitive to etomidate, propofol, midazolam, and isoflurane. Thus they provide a potential substrate for the amnesic properties of anesthetics. These same receptors also contribute to slow phasic (synaptic) currents, which are produced by spillover of GABA from synapses. Their slow time course and location, matching those of synaptic NMDA receptors, place them in an ideal position to modulate synaptic plasticity. Indeed, reducing or eliminating α 5 -GABA A receptors by pharmacologic or genetic means attenuates the amnestic properties of the GABA A receptor-selective anesthetic etomidate. However, since elimination of α 5 -GABA A receptors from pyramidal neurons does not reproduce this effect, modulation of other cell types (interneurons or glia) may also play a role in anesthetic-induced amnesia and other anesthetic end points.
Presynaptic Versus Postsynaptic Effects on Synaptic Transmission
General anesthetics have potent and specific effects on synaptic transmission, including presynaptic actions (by altering transmitter release) and postsynaptic actions (by altering the postsynaptic responses of neurons to specific transmitters). The relative contributions of presynaptic compared with postsynaptic anesthetic effects on synaptic transmission have been difficult to resolve, probably because the effects are transmitter- and synapse-specific. The net effect of anesthetics on synaptic transmission is determined by the relative magnitude and direction of both their presynaptic and postsynaptic effects. The general effects of inhaled anesthetics are to increase inhibitory synaptic transmission and to inhibit excitatory synaptic transmission ( Fig. 19.9 ).

Excitatory synaptic excitation is generally decreased by volatile anesthetics ( Fig. 19.10 ). Experiments in various slice preparations indicate that reduced excitation is primarily caused by presynaptic mechanisms. A postsynaptic mechanism is also involved because the response to directly applied glutamate is reduced to some degree. Volatile anesthetics have inconsistent effects on cloned AMPA or NMDA glutamate receptors, but they potentiate kainite receptors, consistent with a predominantly presynaptic mechanism for glutamatergic synapses. By contrast, the effects of the nonhalogenated inhaled anesthetics (xenon, nitrous oxide, cyclopropane) appear to be mediated primarily by inhibition of postsynaptic NMDA receptors (discussed earlier). Under some circumstances, such as in patients with defects in mitochondrial complex 1 and in mice carrying mutations in mitochondrial complex 1, inhaled agents suppress glutamate release by interfering with the energy-intensive glutamate recycling pathways, thereby leading to extreme anesthetic sensitivity. Recent evidence from conditional knockout mice indicates this mechanism may contribute to various end points even in nonpathogenic states.

Augmentation of GABAergic inhibition by most general anesthetics is mediated by both presynaptic and postsynaptic mechanisms. Enhancement of postsynaptic and extrasynaptic GABA A receptors is well recognized. Volatile anesthetics increase spontaneous GABA release and inhibitory postsynaptic current (IPSC) frequency —that is, their presynaptic effects at GABAergic terminals are distinct from those at glutamatergic synapses.
The mechanisms for the presynaptic effects of inhaled anesthetics, like those for their postsynaptic effects, are complex and involve multiple targets. Although a synapse-specific contribution of presynaptic Ca 2+ channels is likely, presynaptic Na + channels are more sensitive than the Ca 2+ channels coupled to glutamate release. This finding is consistent with observations that the predominant Ca 2+ channel coupled to neurotransmitter release at hippocampal glutamatergic synapses (P/Q-type) is insensitive to isoflurane. Other presynaptic mechanisms have been proposed, including actions on the vesicle fusion process, as demonstrated in the model organism Caenorhabditis elegans . However, isoflurane effects on exocytosis in rat hippocampal neurons occur primarily upstream of vesicle fusion.
Simple Circuits and Complex Networks
Simple Circuit Phenomena
The development of a mechanistic understanding of phenomena involving complex circuits has been greatly facilitated by the study of anatomically reduced (in vitro) or physiologically simplified (in vivo) preparations complemented by computer simulations (in silico). These approaches are essential for integrating reductionist observations of the multiple molecular effects of anesthetics into functional models relevant to behavioral end points. Anesthetic effects have been studied in acute brain slice preparations from various regions of the CNS (hippocampus, amygdala, cortex, thalamus, brain stem, and spinal cord)—most often from rodents. Brain slices preserve native connections but usually lack natural inputs and outputs. Slices from developing mammalian brain can also be cultured in vitro. These “organotypic slice cultures” preserve a high degree of synaptic connectivity and display spontaneous network activity, typically absent from “acute slices.” Simplified in vivo preparations involve phenomena (typically evoked responses) with relatively well-understood circuitry. Computer models and simulations can assist in generating hypotheses for experimental testing and developing hypotheses based on experimental data.
Synaptic Plasticity
Paired-pulse depression and paired-pulse facilitation are examples of short-term plasticity in response to external stimulation. Synaptic inhibition is prolonged by volatile anesthetics in vivo and in vitro, in general agreement with the notion that anesthetics enhance functional inhibition in the CNS. Enhancement of paired-pulse facilitation has been attributed to the presynaptic depressant effect of volatile agents ( Fig. 19.11 ).

LTP, a cellular model of learning and memory, is a use-dependent strengthening of glutamatergic excitatory synaptic connections. Volatile anesthetic effects on LTP depend on the experimental preparation. Halothane, enflurane, and isoflurane do not block LTP induction in vivo, whereas ketamine and the NMDA antagonist CPP do. By contrast, isoflurane blocks LTP in the hippocampal slice by enhancing GABA A receptor–mediated inhibition ( Fig. 19.12 ) or blocking neuronal nicotinic receptors, Long-term depression, a use-dependent weakening of excitatory connections that is effectively a homeostatic counterpart of LTP, is also blocked by isoflurane in vitro. The discrepancy between findings in vivo and in vitro remains unexplained.

Spontaneously Active Circuits
Spontaneous neuronal activity is decreased by volatile anesthetics both in vivo and in cortical brain slices. This effect is largely GABA A receptor-dependent and marked even at low sedative concentrations. Because the cortical brain slices lack subcortical input, these results suggest that volatile anesthetics can cause some effects (e.g., sedation) via direct cortical action. However, changes in raw neuronal firing rates do not provide a precise quantitative measure of higher cognitive function, which is better reflected by the relationship of firing patterns to the strength and phase of ongoing cortical rhythms (see next section). Anesthetic effects have also been tested on the circuitry underlying locomotion, a well-studied central pattern generator. Effects of isoflurane on in vitro lamprey and rat spinal cord preparations support the spinal cord as the primary target for volatile anesthetic–induced immobility.
Rhythms and Simulations
The brain perpetually generates complex electrical rhythms (oscillations in extracellular field potentials) that range in frequency from fractions to hundreds of Hertz (Hz; cycles per second), as recorded on the surface of the scalp as the electroencephalogram (EEG; the higher-frequency oscillations cannot be resolved in surface recordings). All oscillations are behavioral state–dependent and multiple oscillations coexist throughout the sleep-wake cycle. Lower-frequency rhythms allow for integration over longer time periods and typically engage larger areas of the brain. By contrast, higher-frequency rhythms allow for higher temporal resolution on local scales. Cross-frequency modulation allows for integration of both aspects of information processing. Although their physiologic roles are not clear, brain rhythms reflect, subserve, and/or constitute fundamental higher-order processing such that their modulation by anesthetics is of great interest. The current nomenclature of brain rhythms reflects historical conventions and is not based on underlying mechanisms.
δ-Rhythms and Other Slow Rhythms
Oscillations with EEG frequencies from 1.5 to 4 Hz are generally referred to as δ-rhythms, and these oscillations are characteristic of deep sleep and are commonly observed under general anesthesia. Even slower rhythms (below 1 Hz) occur during non–rapid eye movement (NREM) sleep and appear at loss of consciousness induced by propofol and sevoflurane. During natural NREM sleep, δ-rhythms and sleep spindles are phase related to a slower oscillation, suggesting functional interaction. Paroxysmal spindle-like waxing and waning oscillations overriding slower rhythms are also present in the cortical EEG under anesthesia. A change in δ-α phase relationships has been proposed as a “signature” of propofol-induced unconsciousness, but how this comes about, whether similar changes occur for a wider range of anesthetics, and the underlying physiology and functional significance are unknown.
θ-Rhythms
θ-Rhythms, present in various cortical structures but most prominent in the hippocampus, are thought to signal the “online state.” They are associated with sensorimotor and mnemonic functions during waking behavior. One component of the θ-rhythm (type I or atropine-resistant) can be affected by amnestic concentrations of isoflurane as well as by the amnestic nonimmobilizer F6, indicating a potential network-level signature effect for anesthetic-induced amnesia. Type II θ-rhythm (atropine-sensitive) can be evoked under anesthesia and is slowed and potentiated by halothane. Interestingly, this halothane-induced oscillation disappears in TASK-3 knockout mice.
γ-Rhythms
This designation includes an extremely broad and probably functionally and mechanistically heterogeneous spectrum of rhythms. It is frequently subdivided into slow γ (30-50 Hz; i.e., the spectrum above β-rhythms); γ (50-90 Hz), and fast-, ultra-γ, or ε-rhythms (>90 and up to hundreds of Hz). Fast GABA A ergic synaptic inhibition and the intrinsic resonant properties of neurons play important roles in γ-physiology, making these obvious candidates for anesthetic modulation. Isoflurane slows the frequency of evoked γ-oscillations (30-90 Hz, also known as “40-Hz rhythms”) in humans. Studies of γ-oscillations in vitro suggest that their frequency depends on the time constant of decay of GABA A receptor–mediated synaptic currents in inhibitory networks. Isoflurane slows γ-rhythms in hippocampal and neocortical slices to a comparable degree as it does in humans, providing a tentative link between receptor and circuit-level effects. However, the interaction between anesthetics and behaviorally relevant network effects is likely to be complex because flash-evoked γ-oscillations in the primary visual cortex are not affected by inhaled agents, whereas feedback information transfer at γ-frequencies between the visual and frontal cortices is disrupted. Moreover, many brain rhythms are interlinked (e.g., θ-rhythm modulates γ-oscillations (θ−γ nesting). The nature of their modulation by anesthetics as well as its relevance are far from clear.
Models and Simulations
On the macroscopic scale, computer simulations can provide an integrated picture of modulation of dynamic neuronal and network activity. “Bottom-up” neuron-by-neuron approaches are based on computational models of individual neurons, known anesthetic effects on intrinsic and synaptic membrane conductances, and simple network models. Computer simulations of anesthetic effects on integrated outputs can thereby be generated (e.g., the ring of pacemaker neurons). These models obviously rely on the accuracy of the derived characteristics of real neurons and networks as well as knowledge of the effects of anesthetics; the scale of simulations is limited by the complexity of its elements. An alternative is a “top-down” approach, such as mean-field modeling, in which molecular and cellular individual accuracy detail is sacrificed in favor of global dynamics. For example, global cortical phenomena, such as anesthetic-induced seizures, have been modeled as phase transitions based on mean interactions between populations of averaged neurons (analogous to the EEG signal that also averages the signals of neuronal populations). This approach can be extended to other global cortical phenomena such as consciousness. Neuronal modeling and computer simulations may gain importance in the future as bridges between theoretical and experimental studies of anesthesia.
Research Strategies for the Future
The search for anesthetic mechanisms is driven by advances in the basic sciences. Some strategies that should facilitate understanding of anesthetic mechanisms include use of agonists or antagonists in vivo, nonanesthetics or nonimmobilizers, high-resolution imaging of the functioning brain, transgenic animals, and the application of the latest techniques of molecular genetics.
Pharmacologic Approaches
Agonists, Antagonists, and Experimental Anesthetics
Use of receptor-specific agonists and antagonists provides a pharmacologic method for bridging in vitro with in vivo studies. In this approach, a receptor may be tested for its contribution to a specific end point (e.g., immobility) according to the criteria presented earlier. This approach was used to exclude an important role for NMDA receptor block in the immobilizing action of volatile anesthetics but did not yield conclusive results for the roles of GABA A and GlyR for immobilization, probably owing to the intricacies of drug-receptor interactions on different levels of integration in complex networks like the spinal cord. A complementary pharmacologic approach using experimental anesthetics that inhibit NMDA receptors in vitro with different potencies supports the conclusion that NMDA receptor blockade does not contribute significantly to immobility by conventional volatile anesthetics. Refinement of this strategy involves the anatomically discrete application of drugs to nuclei with known function. For example, the tuberomammillary nucleus (part of the endogenous sleep pathway) mediates the sedative component of anesthesia for some intravenous anesthetics (e.g., propofol). A discrete site of general anesthetic action for GABAergic drugs in the mesopontine tegmentum has also been proposed based on this strategy. However, these approaches suffer from the fact that, invariably, high concentrations of drugs have to be injected locally to observe pharmacologic effects (because of rapid redistribution), so that such observations need to be substantiated using more sophisticated approaches, such as genetic manipulations (see later).
Nonimmobilizers
Nonimmobilizers are compounds with physicochemical characteristics similar to those of conventional inhaled anesthetics, but their predicted anesthetizing concentrations (based on their lipid solubility and the Meyer-Overton correlation [MAC pred ]) do not induce immobility. Initially termed nonanesthetics, the terminology was revised when it was discovered that at least some of them cause amnesia at similar MAC pred fractions to classic volatile anesthetics. If a molecular or cellular process is affected in similar ways by an anesthetic and a nonimmobilizer, that process is not relevant for the anesthetic state, with the notable exception of amnesia. Despite this elegant rationale, only a limited number of receptors have been excluded because, compared with volatile anesthetics, nonimmobilizers are relatively target selective. These compounds have the potential to provide insights beyond the initially envisaged receptor-level studies by allowing the separation of sedation from amnesia for the study of underlying network activity in vivo.
Photoreactive Anesthetics
An approach that has proven to be very successful with intravenous drugs is one in which an analog of an anesthetic is synthesized that bears a photoreactive group (often a tri-fluoro-diazirine) which, when irradiated at a wavelength of around 300 nm, reacts irreversibly with some amino acids in the anesthetic binding site. This approach, of course, requires that the pharmacologic profile of the analog is very similar to that of the parent compound, so that the relevant binding site is identified. Photoreactive analogs of inhaled anesthetics have been synthesized and four putative sites on GABA A receptors have been identified. . One advantage of this approach over x-ray crystallography is that anesthetic binding to different conformational states of the receptor are, at least in principle, more accessible to analysis.
Genetic Approaches
Whole-Organism Genetics
Genetic strategies on whole organisms take two forms: forward and reverse. The reverse genetic approach focuses on a particular gene chosen because there are reasons to hypothesize that its product may be important to anesthesia. Examples of this strategy are targeted mutations that alter the sensitivity of specific neurotransmitter receptors to anesthetics. Initially, these mutations were used to identify anesthetic binding sites. Subsequently, transgenic animals rendered resistant to anesthetics, either by deletion of a putative target protein from the genome or by expressing a target receptor engineered to be insensitive to an anesthetic, were used to test behavioral relevance of the altered gene product for the production of anesthesia. Forward genetics, by contrast, is a discovery process that involves the study of randomly generated mutations (either experimentally induced or naturally occurring polymorphisms) that affect the phenotype of interest (i.e., anesthetic end points) in a population. This approach, however, has not been widely used.
Molecular Genetics
A third genetic approach that is playing an increasing role in neuroscience is the selective modification of neuronal function using molecular genetics. Many different approaches are used, ranging from the selective knockdown of specific receptor populations using microRNA (a small noncoding piece of RNA that can selectively reduce protein expression), the block of neurotransmitter release from a population of neurons using tetanus toxin light chain, and the selective activation or inhibition of neuronal networks using optogenetics or pharmacogenetics. A powerful complement to these techniques is the ability to deliver artificial transgenes to defined neuronal populations using adeno-associated virus (AAV), and mouse lines that express Cre recombinase in particular cell types, which allow the selective expression of those genes in those specific cells. These approaches are only just beginning to be used to identify neuronal pathways that are involved in anesthetic action. For example, it has recently been shown that the sedative effects of propofol require the activation of the lateral habenula, an excitatory nucleus that lies close to the thalamus. These approaches are likely to have an increasing impact on our understanding of the mechanisms of inhaled general anesthetics.
Knockout and Knockin Animals
In the knockout approach, expression of the gene encoding a protein of interest is disrupted by a specific deletion or insertion. Nearly all such studies have been carried out in mice. A well-recognized problem with the global knockout approach is that extensive compensatory changes can be induced, from anomalies that are lethal in utero to insidious (but experimentally confounding) influences that might be expressed only at maturity. A complementary strategy is the conditional knockout, in which the genetic deletion is restricted either anatomically (limited to certain brain regions) or temporally (at a known point in time). These strategies can minimize developmental anomalies and reduce the likelihood of compensatory changes. In the knockin approach, a mutation, usually of a single amino acid residue, is targeted to produce a protein with altered sensitivity to a drug of interest. Ideally, this mutation remains completely silent in the absence of the drug; that is, it does not perturb the normal expression and function of the protein of interest or alter the expression of other genes.
GABA A receptors
Results from transgenic animals illustrate both the utility and the difficulties of the genetic approach with respect to inhaled agents. The conditional forebrain-restricted GABA A receptor α 1 -subunit knockout mouse was found to be less sensitive to isoflurane-induced amnesia than wild-type mice, which led to the conclusion that action at these receptors contributes to isoflurane’s amnesic effects. By contrast, a mouse harboring a mutation of the GABA A receptor α 1 subunit that renders the receptor insensitive to isoflurane in vitro did not show reduced sensitivity to either the amnesic or the immobilizing effects of isoflurane, leading to the conclusion that this subunit does not mediate the impairment of learning and memory by isoflurane. Similar experiments indicate that action at the GABA A receptor β 3 subunit does not mediate immobility or amnesia by isoflurane. This bottom-up genetic approach is a work-intensive but powerful tool that has yielded clear results with the receptor-specific intravenous anesthetics, but it has proved challenging to apply it to the more promiscuous inhaled agents.
Glycine α1–containing receptors
Pharmacologic studies supported the notion that glycinergic neurotransmission might be the effector for the immobilizing action of inhaled anesthetics in the spinal cord, where glycine replaces GABA as the principal inhibitory transmitter. However, mice harboring mutations that render α 1 subunit–containing glycine receptors largely insensitive to alcohol and inhaled ether anesthetics did not demonstrate a concordant change in MAC values. Because α 1 is the most widely expressed subunit in adult animals, it is unlikely that action at glycine receptors plays an important part in the immobilizing action of inhaled anesthetics.
Two-pore domain K+ channels
Use of mice harboring knockout mutations of several two-pore domain K + channel (K 2P ) family members (TASK-1, TASK-3, TREK-1) has demonstrated a role for these channels in volatile anesthesia. For example, TREK-1 knockout mice are partially resistant to all volatile anesthetics tested with respect to both loss of righting reflex (a surrogate measure of consciousness) and immobility, but anesthesia can still be induced, albeit at higher anesthetic concentrations. Interestingly, responses to pentobarbital are unaffected, indicating that the mutation does not cause a generalized resistance to anesthesia.
Forward and Population Genetics
The nematode C. elegans and the fruit fly Drosophila melanogaster , with 302 and 100,000 neurons, respectively, have also been used as model organisms in anesthesia research. Mutations in a number of C. elegans genes affect sensitivity to volatile anesthetics, most notably unc-1 , a close homologue of the mammalian protein stomatin. Yeasts have also been used as model organisms, with even more obvious limitations with respect to identification of appropriate anesthetic end points.
Sensitivity to anesthetics is a quantitative trait (varying continuously in a population). Quantitative genetics is the study of the heritability of continuous traits. These traits are controlled by genes represented in quantitative trait loci (QTLs). A top-down population-based approach has been used to localize QTLs that govern susceptibility of individuals to anesthetics, in higher and lower organisms. Starting from the observation that inbred mouse strains vary in their sensitivity to isoflurane, microsatellite-based linkage analysis and, alternatively, single-nucleotide polymorphism–based analysis of genetic variation localized a QTL for isoflurane immobilization to the proximal part of mouse chromosome 7. Genetic variability in the sensitivity of D. melanogaster to inhaled anesthetics has also been demonstrated. This type of analysis promises to assist in defining the genetic basis for variability in the susceptibility to both primary anesthetic end points and side effects.
Functional Imaging and High-Density Electroencephalography
Identification of the anatomic and functional substrates for anesthetic effects on consciousness, memory, and immobility is now approachable with improved imaging techniques. Imaging is based on mapping of either metabolic or hemodynamic changes as surrogate measures of neuronal activity, as in positron emission tomography (PET) and functional magnetic resonance imaging, or on mapping electrical activity with high-density EEG, magnetoencephalography, and low-resolution brain electromagnetic tomography. Properties of receptors can also be probed with radioactive ligands by PET. These techniques have the capacity to identify neuroanatomic substrates of drug action with method-specific limitations. Results from functional PET imaging suggest that propofol suppresses episodic memory by targeting the prefrontal and posterior parietal cortex as opposed to the medial temporal lobe and that suppression of consciousness is caused by anesthetic action in the thalamus, parts of the medial and posterior parietal cortex, and/or the posterior cingulate and medial parietal cortex. Although observations of regionally specific and global suppressive effects of anesthetics on metabolic activity are unlikely to provide a definitive mechanistic understanding, such information can facilitate hypotheses and experimentally testable predictions.
Advanced analytical approaches based on theories from mathematical and statistical sciences are being increasingly applied to enhance the power of existing technologies. Magnetic resonance images and high-density EEG recordings of the brain reveal strong interregional connections, but the considerable potential of this connectivity information to better understand the brain’s response to anesthesia has only recently begun to be tapped. The growing use of invasive recording techniques (e.g., brain surface electrode grids and microelectrodes implanted deep into the brain for functional neurosurgical therapies) is also advancing the frontiers of neuroscience in general and the understanding of anesthetic mechanisms in particular.
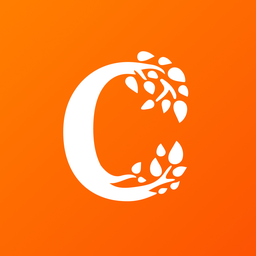
Full access? Get Clinical Tree
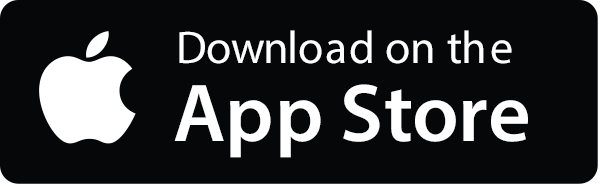
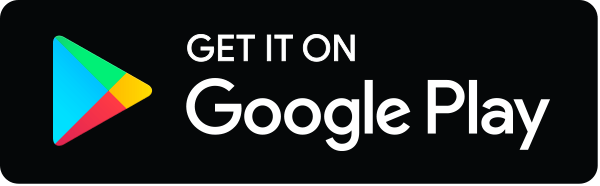