Chapter 8
Inhalation Anesthetics
In the span of almost two centuries major advances have been made in the development and clinical use of inhalation anesthetics (Table 8-1); this continuum includes the investigation of nitrous oxide (N2O) in 1800 by Humphry Davy1 following its preliminary discovery by Dr. Priestly in 17862 with what was termed “dephlogisticated air.” Then 195 years later the most current inhaled anesthetic (sevoflurane) was approved by the Food and Drug Administration in 1995. Interestingly, each of these agents share a common characteristic that promotes their clinical use today—a low blood:gas solubility coefficient, which portends a favorable pharmacokinetic profile.
TABLE 8-1
History of the Introduction of Inhalation Anesthetics
Anesthetic | Year(s) Anesthetic Properties Demonstrated/Introduced |
Ether (Crawford Long) | 1842 |
N2O (Horace Wells) | 1845 |
Chloroform (James Simpson) | 1847 |
Cyclopropane (George Lucas, Velyien Henderson) | 1934 |
Fluroxene | 1951 |
Halothane | 1956 |
Methoxyflurane | 1960 |
Enflurane | 1973 |
Isoflurane | 1981 |
Desflurane | 1993 |
Sevoflurane | 1995 |
The present-day use of N2O can be credited to Edmund Andrews, a professor of surgery in Chicago. In 1868 he declared that a safer anesthetic could result from combining oxygen (O2) with N2O.3 Before that time N2O was administered through a mouthpiece with a nose clamp to prevent the rebreathing of air.
One of the earliest “complete” anesthetic agents used was diethyl ether (C2H5–O–C2H5). The first ether anesthetic was administered in Georgia on March 30, 1842, when Crawford Long anesthetized a patient for a minor operation (excised a tumor).4 However, the recognition of numerous unfavorable characteristics (excessive secretions with inhalation induction, laryngospasm, excessive depths of anesthesia) prompted its disappearance from clinical practice as newer agents were subsequently developed.
In the 1930s, research into potential anesthetic agents was based on the principle of a structure-activity relationship.5 One of the earliest inhalation anesthetics developed in this manner was divinyl ether. Halothane was introduced into clinical practice in 1956 by Bryce-Smith and O’Brien in Oxford6 and Johnstone in Manchester7 and represented a significant advancement in inhalation anesthesia. Its sweet odor, nonflammability, and high potency offered clinical characteristics that were absent from the previously available inhaled anesthetics. The search for newer and improved inhalation anesthetics persisted as concerns with hepatotoxicity and arrhythmogenicity of this alkane derivative began to be documented.
Relationship of Chemical Structure and Agent Characteristics
An understanding of the chemical structure of inhalation agents provides insight into their physical properties (e.g., flammability). However, the relationship between the pharmacologic characteristics (e.g., arrhythmogenic properties) and chemical structure of agents is not as predictable. This section reviews the structure-activity relationship of anesthetic vapors and their clinical relevance. Some selected nomenclature in regard to physical and chemical properties are listed in Table 8-2.
All commonly used inhalation agents are ethers (R–O–R) or aliphatic hydrocarbons (straight-chained or branched nonaromatic hydrocarbons) with no more than four carbon atoms (Figure 8-1). The length of the anesthetic molecule is significant in that immobility (anesthetic effect) is attenuated or lost if carbon atom chain length exceeds a distance of four or five carbon atoms (5 angstroms [Å]).8 The molecular shape of the agents is spherical or cylindrical with a length less than 1.5 times the diameter.9
Anesthetic potency has been shown to increase when a halogen with a lower atomic mass unit (amu) is replaced by a heavier halogen (e.g., bromine at 80 amu substituted for fluorine at 19 amu).10–12 Nonetheless, a ceiling effect exists with halogenation of anesthetic compounds. For example, adding F atoms to ether results in a continuum in which the ether becomes more potent, then acts as a strong convulsant, and finally changes to an inert compound with full fluorination.13
With regard to arrhythmogenic properties, increasing the number of halogen atoms within a volatile agent favors the genesis of cardiac dysrhythmias.13 Nevertheless, alkanes that contain five halogens (e.g., halothane) are more prone to induce arrhythmias than ethers with six halogen atoms (e.g., isoflurane).14
Flammability is reduced and chemical stability enhanced by substituting hydrogen atoms with halogens. The epitome of this relationship is demonstrated with desflurane, a compound that contains fluorine as its only halogen and thus strongly resists biodegradation; desflurane is metabolized one tenth as much as isoflurane.15,16
Metabolism
As stated previously, the chemical structure of each inhalation agent determines the extent to which it is metabolized. In general, increasing the number of fluorine atoms to a anesthetic molecule retards biodegradation (halothane has 3 and desflurane has 6). The current agents undergo metabolism at the following rates: sevoflurane 5% to 8% and isoflurane, desflurane, and nitrous oxide in trace amounts.17–20 Desflurane has been shown to resist biodegradation, even after 7.35 MAC-hours, as determined by peak mean urinary excretion rate of trifluoroacetic acid (TFA). This metabolite (TFA) is recognized as being a sensitive marker of desflurane metabolism.21
Pharmacodynamics
• Lipid solubility is directly proportional to potency (Meyer-Overton rule).22,23
• Reversal of anesthetic effect can be achieved with the application of pressure, with some exceptions (species variation).24
• No common chemical structure for the variety of compounds is capable of producing anesthesia.
• The molecular and structural changes responsible for producing anesthesia must occur within seconds and be reversible.
• A reduction in body temperature lowers anesthetic requirements.
The unitary hypothesis was previously offered as an attempt to meet these prerequisites. This theory proposed that all inhalation anesthetics work via a similar (undefined) mechanism of action but not necessarily at the same site of action. One factor that supported this hypothesis was the Meyer-Overton correlation, which recognized the more lipid soluble the agent, the greater its potency (the lower its MAC value). This correlation suggested that anesthesia is produced by the volume of anesthetic molecules present (dissolved) at the site, not by the type of inhaled agent present. Nevertheless, continued research on the mechanism of action of inhalation anesthetics has mitigated the utility of this theory.25
In vitro, in vivo, animal, and human subject research has permitted the description of the mechanism of action of inhaled anesthetics relative to distinct anatomic regions of the body (including the cellular level) and molecular changes. The spinal cord is known to mediate immobility to a painful stimulus via several mechanisms including (1) enhancing background potassium (K+) currents in tandem-pore-domain, weak inward-rectifying K+ channels (TWIK, TASK [TWIK-related acid-sensitive K+ channel])26–28 and (2) reducing spontaneous action potential firing of spinal neurons via glycine receptors and γ-aminobutyric acid type A [GABAA]) receptors.29 Investigators have also demonstrated that nonimmobilizers with lipophilic characteristics (e.g., perfluoropentane) are able to produce amnesia but not immobility to noxious stimuli, which suggests two separate sites and mechanisms of anesthetic action for some drugs.30 In contrast with other research, spinal and cerebral GABAA receptors (same receptor-clinical effect) were shown to contribute to volatile anesthetic’s ability to produce immobility; therefore the anesthetic effect of immobility is modulated at the spinal cord and supraspinal level.31,32 In summary, research has validated the concept of a distinct anatomic division for the mechanism of action of volatile agents as being oversimplistic (and inaccurate); one example being the isolation of TWIK and TASK (members of the tandem-pore-domain K+ channel subfamily) in both the spinal cord and brain.27, 28 Clearly, supraspinal areas of the nervous system are recognized to mediate amnesia and immobility.28,32–35
Other specific anatomic sites where volatile anesthetics produce an effect include the reticular formation within the brainstem,36 cerebral cortex,37 and hippocampus.38 Evidence of changes in cortical activity by volatile agents includes alterations in electroencephalogram (EEG) activity. All inhalation agents cause a dose-dependent change in the EEG—an initial increase in voltage (and decrease in frequency), then a peak, followed by a decline.39,40 Deeper levels of anesthesia produce burst suppression and eventually a flat EEG.41 Nonspecific generalized EEG changes may also persist for several days postoperatively.42
Increased or decreased neuronal excitability and enhanced or depressed inhibitory postsynaptic currents can occur, depending on which anesthetic agent or specific area within the central nervous system (CNS) is manipulated. In addition to supraspinal effects, depression of motor neurons within the spinal cord has also occurred with volatile anesthetics.43,44
On a molecular level, researchers have found that the most likely site of action for volatile anesthetics involves interactions with membrane proteins in specific receptors (stereoselective) and not perturbation of lipid bilayers.45–48 The primary receptor within the CNS believed to modulate anesthetic effects is the GABA receptor, specifically the subtype A.31,33,34,49 This receptor is located abundantly in the CNS and is a ligand-gated chloride (Cl−) ion channel.50 Agonism of this receptor by full anesthetics (volatile agents) results in enhanced Cl− conductance,51 which leads to inhibitory actions on local neurons.50 Ultimately, what is expressed is an extension of the amount of time the Cl− channel remains open. Neuronal nicotinic acetylcholine receptors (nAChRs) have also been shown to be highly sensitive to inhalational anesthetics and are believed to significantly influence several stages of anesthesia.52
An investigation by Kaech et al.53 revealed a new anesthetic site of action within the CNS; chloroform, diethyl ether, methoxyflurane, halothane, enflurane, and isoflurane were each shown (in clinically relevant concentrations) to block the morphologic plasticity of dendritic spines. Prior research has demonstrated that dendritic spines can change shape in seconds.54 This phenomenon occurs secondary to motile actin, which is abundant in the spines.55 These volatile agents were found to strongly inhibit actin motility, which blocked changes in dendritic spine shape; the inhibition was fully reversed after removal of the agents. The dendritic spines serve as excitatory postsynaptic contact sites.56 They are extremely abundant in the cerebral cortex (greater than 1013) and are also located in large numbers in the cerebellum, basal ganglia, and olfactory bulb.57 The details of how these rapid morphologic changes in dendritic spines contribute to an anesthetic state are unclear and merit further research.
The CNS effects of amnesia and loss of consciousness likely are produced separately from the immobility conceptualized in the theory of MAC. The concept of MAC refers to the concentration required to prevent movement in response to a surgical situation. This is probably the result of an effect at the spinal cord level via glycine, sodium, and N-methyl-d-aspartate receptor (NMDAR) action. Potassium, GABAA, opioid, α2−, 5HT3, and acetylcholine receptors are likely not involved in producing immobility.58 They may be involved in varying degrees in the amnestic and anesthetic effects in the CNS. Lastly, researchers have found halogenated inhaled anesthetics inhibit voltage-gated sodium (Na+) channels, which may represent a common means for producing an anesthetic effect.59 A summary of reviewed mechanisms of action of inhalation anesthetics is provided in Box 8-1.
Minimum Alveolar Concentration
A useful means of comparing the potencies of inhalation agents is to use the concept of MAC, defined as the minimum alveolar concentration at equilibrium (expressed as a percentage of 1 atmosphere) in which 50% of subjects will not respond to a painful stimulus (i.e., initial surgical skin incision).60 The response is defined as gross, purposeful movement of the head or extremities. The MAC values for the modern inhalation agents are listed in Table 8-3.61–64
TABLE 8-3
Potencies of Volatile Anesthetics in Humans with and without N2O Expressed in MAC Values
MAC, Minimum alveolar concentration; N2O, nitrous oxide.
The MAC of volatile agents can be affected by numerous factors, surprisingly even hair color.65,66 With increasing age, the MAC of all inhaled anesthetics is reduced; in humans with mean age 18 to 30 years, the MAC of desflurane is 7.25%, in contrast to 6% in humans 30 to 55 years of age.63 Infants represent an exception to the MAC age concept in that their anesthetic requirements exceed those of neonates. In general, the duration of anesthesia is believed not to effect MAC; however, one group of investigators found the MAC of isoflurane was reduced over time during surgery.67 Box 8-2 lists variables shown to reduce and increase MAC.
Two other areas related to MAC are MAC-awake and MAC-BAR (block adrenergic response). MAC-awake is defined as the minimum alveolar concentration at which 50% of subjects will respond to the command “open your eyes.” It has also been described as the anesthetic concentration that is between the end-tidal values that allow and prevent response to a command.68 This end-tidal concentration is usually associated with a loss of recall and encompasses approximately one third of MAC values. MAC-awake can also be used in combination with MAC values to evaluate the potency of each agent with regard to amnestic properties. This is done by dividing MAC-awake by MAC (MAC-awake/MAC ratio). This parameter indicates that agents with ratios between 0.3 and 0.4 (e.g., desflurane, sevoflurane, isoflurane) are considered potent anesthetics. In contrast, N2O, which has a ratio of 0.64, is considered a weak amnestic agent.
The MAC-BAR parameter represents the MAC necessary to block the adrenergic response (e.g., changes in plasma norepinephrine concentration, heart rate [HR], rate-pressure product, and mean arterial pressure) to skin incision. It can be expressed as a MAC-BAR50 or MAC-BAR95. The former is similar to AD95 values, which represent the anesthetic dose that inhibits somatic evidence of light anesthesia in 95% of subjects in response to skin incision. Established MAC-BAR50 values for volatile agents include (in 60% N2O) 1.85 MAC for both isoflurane and desflurane. Desflurane’s MAC-BAR50 value has been shown to be reduced by 85% with the use of 1.5 mcg/kg of fentanyl given IV 5 minutes before skin incision.69 When investigating desflurane in 60% N2O combined with a remifentanil at a target plasma concentration of 1 ng/mL (via a computer-driven infusion device), the MAC-BAR50 is reduced by 60% and with a target concentration of 3 ng/mL further reduced by 30%.70
The MAC-BAR50 for sevoflurane in 66% N2O is 2.2.71 In canine studies it has been shown to be reduced (but not in a dose-dependent manner) with intravenous ketamine.72 It should be emphasized that MAC-BAR values exceed the requirements for ablation of skeletal muscle movement with surgical stimulation; therefore blocking an adrenergic response requires a greater depth of anesthesia than preventing skeletal muscle movement. From a clinical standpoint, patients usually require anesthetic concentrations that exceed MAC by 20% to 30% (1.2 to 1.3 times MAC). At this alveolar concentration, somatic evidence of light anesthesia will commonly be abated, and fewer patients will respond adrenergically to the stresses of surgery.73
The concept of MAC has limitations when applied clinically to determine adequacy of anesthesia. It should be viewed as a general guide to the overall depth of anesthesia. One variable that restricts its application is the frequency at which surgical patients receive muscle relaxants, which attenuates the recognition of skeletal muscle movement in response to light planes of anesthesia. This results in the dependence of anesthesia providers on other traditional signs of anesthetic depth, such as changes in heart rate (HR), blood pressure, pupillary size, and sweating. Unfortunately, a light plane of anesthesia can exist even with a decreased blood pressure and a normal heart rate (e.g., patients with limited cardiac reserve). Pupillary changes can also be affected by opioids (miosis) and volatile agents (mydriasis) over time, even in the absence of surgical stimuli.74 Also, the usefulness of a traditional clinical end-point as a guide to depth of anesthesia can change over time. For example, one investigator found that decreases in blood pressure served as an estimate of anesthetic depth during the first hour of an anesthetic, but after 5 hours they were unreliable—further declines in blood pressure did not occur even with increasing concentrations of halothane.75 The challenge to the anesthesia provider is to estimate anesthetic depth based on a collation of variables (HR, blood pressure, synergistic and additive effects of anesthetic adjuvants, volume status, physiologic reserve, MAC, MAC-BAR, and MAC intubation values). The last variable (MAC intubation) is similar to MAC-BAR in that its values exceed the anesthetic requirements for surgical skin incision. Clearly, different stimuli require different end-tidal concentrations (brain anesthetic partial pressures) of volatile anesthetics.76
Influence of Inhalation Agents on Organs and Systems
Cerebral Metabolic Rate and Cerebral Blood Flow
In general, volatile agents decrease cerebral metabolic rate of O2 consumption (CMRO2) in a dose-dependent manner, whereas their effect on cerebral blood flow is variable; the latter has been reported by various researchers to be unchanged,77,78 increased (dose-dependent and time-dependent manner),79–81 or decreased.82 When vascular resistance is decreased, CBF, cerebral blood volume (CBV), and CSFP increase. The order of potency for increasing CBF varies; it is affected by the dose of volatile anesthetic,81,83 the administration of other drugs (e.g., propofol, N2O),84 the rate of change in end-tidal concentration of agent,79 and the animal model used.85 In other cases, differences in research findings lack a plausible explanation. A distinct picture of a homogeneous versus heterogeneous change in CBF, CMRO2, and other anesthetic effects of volatile agents has been noted.86,87 For example, in rats halothane has been shown to globally increase CBF, whereas isoflurane’s sphere of influence predominates in the subcortical regions and hindbrain structures.88
Uncoupling of Cerebral Blood Flow and Metabolism
When decreases in CMRO2 are accompanied by increases in CBF, uncoupling is said to occur. As noted earlier, volatile anesthetics are capable of producing this effect. This paradoxic response (decreased CMRO2 occurring in conjunction with increased CBF) seems not to occur with 1.0 MAC or less of halothane and isoflurane89; the magnitude of change is variable and dose dependent,81 meaning some flow-metabolism coupling mechanism is preserved.90, 91
Nitrous oxide reduces cerebrovascular tone significantly. This effect is unmasked and enhanced when N2O is combined with a volatile anesthetic (decreased autoregulation).92 The mechanism for increased CBF may be related to a sympathoadrenal-stimulating effect of N2O. The changes produced by N2O in the CMRO2 are the reverse of what takes place with volatile agents (i.e., increased CMRO2).93 Thus N2O increases CMRO2 and CBF. Nevertheless, the combination of elevated CBF and CMRO2 still results in an uncoupling between flow and metabolism, because the increase in CMRO2 exceeds, albeit slightly, the elevation in CBF.94 In summary, N2O use in neurosurgical procedures is acceptable, as long as the anesthesia provider recognizes that its vasodilatory effects might adversely affect surgical outcome in patients with reduced intracranial compliance. Mild hyperventilation to low normal ranges of carbon dioxide helps attenuate the increase in CBF that accompanies the use of N2O.95
Cerebral Vasculature Responsiveness to CO2
The normal physiologic response of the cerebral vasculature to CO2 is to vasoconstrict in the presence of hypocapnia and vasodilate with hypercarbia. This reflex is effective in the acute setting when used during neurosurgical procedures to counteract drug-induced vasodilation and to reduce brain bulk within a closed compartment (cranial vault).96 The usual goal for patients in which a reduction in intracranial volume is desired is a Paco2 of 30 to 35 mmHg with a duration of effectiveness being perhaps no more than 4 to 6 hours.97
Differences exist among the volatile agents in their ability to interfere with the cerebral vasculature’s responsiveness to CO2. Variables that affect the reported differences include the type of surgical procedure the patient is undergoing, associated pathophysiology, and the presence of any coexisting disease(s). For example, patients with hypertension given 1 MAC isoflurane with 67% N2O have better control of CBF via manipulation of arterial CO2 than those receiving 1 MAC sevoflurane with 67% N2O.98 Similar results have been reported by other investigators.99 In contrast, CO2 reactivity in insulin-dependent patients is equally impaired by 1 MAC isoflurane and 1 MAC sevoflurane, each given with 67% nitrous oxide.100
On a side note, sevoflurane has been shown (in rodents) to impair glucose metabolism and produce hyperglycemia.101 Other investigators have suggested that sevoflurane is less vasoactive than halothane, isoflurane, and desflurane and recommend it as a good alternative to propofol in patients with normal intracranial pressure.102 It has also been reported to better preserve dynamic cerebral autoregulation than isoflurane when both are given at 1.5 MAC in combination with 100% O2.103 Desflurane administered to patients undergoing craniotomy for tumor resection with an air-O2 mixture at 1.0 to 1.5 MAC has been shown to act similarly to isoflurane and maintain cerebrovascular reactivity to CO2 and cerebrospinal fluid pressure.104–106 These research findings suggest that increases in CBF produced by isoflurane, desflurane,106 and sevoflurane107 can be effectively prevented by very mild hyperventilation and using concentrations less than 1.5 MAC. In addition, anesthesia consisting of sevoflurane and remifentanil appears to be similar to total intravenous anesthesia (TIVA [i.e., propofol combined with remifentanil]) during supratentorial craniotomy procedures in regard to emergence times, adverse effects, intraoperative hemodynamic events, and brain relaxation scores.108
Electroencephalogram and Evoked Potentials
The volatile agents produce a dose-related suppression of EEG activity (initial increase [later a decline] in amplitude and decreased frequency) and at high concentrations produce electrical quiescence.109 At deeper levels of anesthesia, the EEG may temporarily stop recording; at such time, burst suppression is said to have occurred.
For those procedures requiring monitoring of the integrity of the spinal cord or mapping of cortical regions of the brain, the anesthetist should be aware that inhalation agents can skew cortical somatosensory, motor, brainstem, auditory, and visual evoked potentials. Isoflurane, desflurane, sevoflurane, and N2O produce a dose-dependent reduction in these evoked potentials, with visual evoked potentials being most sensitive and brainstem-evoked potentials most resistant.109,110 Two evoked-potential variables commonly assessed are latency and amplitude. An increase in latency or decrease in amplitude of evoked potentials can reflect ischemia or be secondary to the volatile agent. Latency is the time between the initiation of a peripheral stimulus (e.g., electrical stimulation of the median nerve at the wrist) and onset of the evoked potential (e.g., cortical) recorded by scalp electrodes.
Isoflurane has been shown to interfere with the recording of cortical somatosensory evoked potentials (cSSEP) at light planes of anesthesia (0.5 MAC with 60% N2O )111 and desflurane and sevoflurane have been found to produce fewer changes than isoflurane in amplitude reduction of cSSEP at 0.7, 1.0, and 1.3 MAC (without N2O). In contrast, no difference exists among the volatile anesthetics’ effect on latency.112 The addition of N2O to isoflurane, desflurane, and sevoflurane can also produce a significant reduction in the amplitude of cSSEPs.113,114 It may be prudent to avoid the use of this agent in patients who have baseline low-amplitude evoked potentials.
Sevoflurane,115,116 unlike desflurane117 and isoflurane,39 can predispose pediatric and adult118 patients to epileptic activity, even though sevoflurane119–121 can suppress drug-induced convulsive activity in a manner similar to desflurane122 and isoflurane.122 Sevoflurane combined with N2O has produced epileptiform EEG activity during inhalation induction with adults in a single-breath technique. A hyperdynamic response can accompany the EEG changes if concurrent hyperventilation occurs. The incidence of epileptiform EEG changes has been shown to nearly double in the presence of hypocapnia (100% versus 47%).123 Similar results have been observed in children aged 2 to 12 years.116 In contrast, intravenous induction with thiopental followed by anesthetic maintenance with 2% end-tidal sevoflurane in air does not produce seizure-like changes in the EEG in children.115 Epileptiform activity has also been reported to occur during emergence from sevoflurane.124
Emergence and Neurologic Assessment in Adults
Although the objective of a smooth and rapid emergence from a general anesthetic is desirable for all surgical patients, it is especially meaningful for neurosurgical candidates. Delayed emergence in this specialty of anesthesia can have devastating consequences. A slow return of consciousness makes it difficult to perform the initial postoperative neurologic examination. It can also add to unnecessary therapeutic or diagnostic intervention and predispose the patient to respiratory complications.125
Because of this, the bias of some anesthesia providers is to administer TIVA techniques such as propofol with remifentanil and/or dexmedetomidine for neurosurgical procedures involving supratentorial tumors. Some investigators report a more rapid awakening (after approximately 2 hours of anesthesia) from TIVA in non-neurosurgical patients compared with sevoflurane and desflurane combined with N2O.126 Recovery profiles for sevoflurane and desflurane indicate they are superior to isoflurane.127,128 In side-by-side comparisons of desflurane and sevoflurane, desflurane permits for a more rapid awakening than sevoflurane in volunteers after 8 hours of exposure.129 In contrast, sevoflurane allows for acute changes in vaporizer settings without evoking neurocirculatory excitation (i.e., significant increases in sympathetic nerve activity, norepinephrine concentrations, heart rate, and mean arterial blood pressure); of particular interest was the finding that desflurane’s sympathomimetic response occurred in response to a controlled adjustment in vaporizer settings (i.e., changing from 6% to 9%)—that is, in the absence of overpressurization.130 Sevoflurane may be preferred over desflurane if concentrations equal to or in excess of 1 MAC are used during neurologic surgery.79 One study also found no difference in early postoperative recovery and cognitive function between a balanced sevoflurane-fentanyl technique versus propofol-remifentanil (TIVA) management in patients undergoing supratentorial intracranial surgery.131 Similarly, a multicenter randomized controlled trial of patients scheduled for elective supratentorial craniotomy surgery found no significant difference between sevoflurane-remifentanil and propofol-remifentanil techniques.108 Further research will help to illuminate whether any substantive differences exist in neurosurgery outcome secondary to the choice of inhaled anesthetic or primary TIVA techniques employed.
An area of ongoing research has been the application of volatile anesthetics via preconditioning and postconditioning techniques for neuroprotection following global and/or focal ischemia/hypoxia.132 One of the etiologies of neurodeficits occurring in this setting is ischemia/reperfusion injury. In vitro and in vivo (animal) cerebral, spinal, and cardiac studies have demonstrated that isoflurane, and especially sevoflurane, can reduce cerebral infarct volume, improve learning and memory deficits, attenuate ischemia/reperfusion injury to the spinal cord, and lessen hypoxia-induced neuronal cell damage.133–137 Problematic with transitioning from bench research to clinical practice is recognizing that the application of inhaled anesthetic preconditioning treatments would require a need to predict when a reperfusion insult would occur; in contrast, volatile anesthetic postconditioning interventions would ideally occur when the patient develops clinical symptoms.
Several foundational questions remain unanswered regarding anesthetic conditioning with humans: What is the ideal time and duration of anesthetic prior to postconditioning? Also, what is the optimal anesthetic dose for producing a neuroprotective effect? Current research suggests that a dose-response relationship and ceiling effect do exist. For example, in one study it was demonstrated that 1.0 MAC sevoflurane reduced infarct size in the setting of reperfusion injury, whereas 0.75 MAC sevoflurane had no effect138; in a second study it was shown that 1.0 MAC and 1.5 MAC sevoflurane given at the start of reperfusion was neuroprotective (focal cerebral ischemia) and yet at 0.5 MAC, no protection was seen.135 Current research suggests that anesthetics can provide long-term durable protection against ischemic injury that is mild to moderate in severity. Experimental data do not provide support for the premise that anesthetics reduce injury when the ischemic injury is severe.139 Anesthetics consistently and meaningfully improve outcome from experimental cerebral ischemia, but only if present during the ischemic insult.140 To summarize, although research continues to help define the future role of inhaled anesthetics in the context of reperfusion injury, currently there is insufficient evidence to definitively recommend the use of one agent over another in the operating room setting.141
Emergence Phenomenon in Children
Sevoflurane and desflurane are associated with emergence agitation or delirium in children.142 The emergence phenomenon is short-lived but troublesome, and the etiology is uncertain. A variety of factors have been suggested to play a potential role in emergence delirium (ED). Restless behavior upon emergence causes discomfort to the child, postanesthesia recovery nurses, and parents. Some suggested factors related to transient emergence agitation are noted in Box 8-3. These children may require analgesics or sedatives, which can delay discharge. Emergence delirium is self-limiting and devoid of apparent sequelae, as long as the child is protected from self-injury. Preventive measures include reducing preoperative anxiety and postoperative pain and providing a quiet, stress-free environment for postanesthesia recovery. Treatment may include small doses of fentanyl, clonidine, or dexmedetomidine. Reuniting the child with the parents is also helpful.
Cardiovascular System
Systemic Hemodynamics
Isoflurane, desflurane, and sevoflurane all reduce mean arterial pressure (MAP) (Figure 8-2) and cardiac output (CO) and cardiac index (CI) in a dose-dependent fashion.130,143–145 The mechanism by which each accomplishes this varies. For example, desflurane, sevoflurane, and isoflurane predominantly reduce MAP via a reduction in systemic vascular resistance (SVR), with the dose-response relationship being least with sevoflurane (Figure 8-3).130,146 Halothane, by comparison, causes less disruption in inherent vascular tone and therefore predominantly reduces MAP by direct myocardial depression versus a reduction in preload.147 Nitrous oxide activates the sympathetic nervous system and increases SVR,148 which can also lead to an increase in central venous pressure (CVP) and arterial pressure. This sympathetic nervous system response appears to be intact during coadministration of volatile agents.144
In general, N2O used in combination with inhalation agents increases SVR and helps support arterial blood pressure. In contrast, with opioids, the addition of N2O augments cardiac depression instead of supporting it149 because N2O also produces a direct negative inotropic effect. This property can be unmasked in patients with decreased left ventricular function secondary to coronary artery disease or valvular heart defects.150 Desflurane supports CI better than halothane at both low and high MAC levels (i.e., 1.66 MAC)151 (Figure 8-4
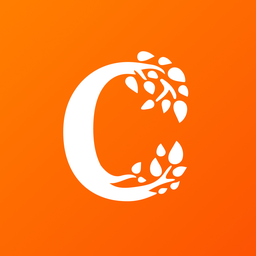
Full access? Get Clinical Tree
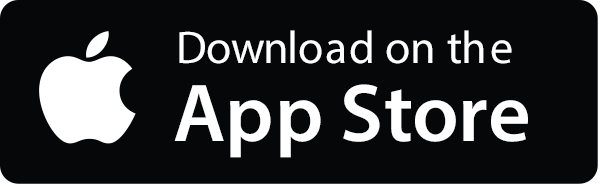
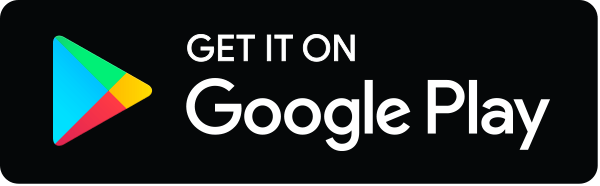