Imaging Pain
Irene Tracey
Martin Ingvar
OBJECTIFYING THE PAIN EXPERIENCE
Over the past 15 years, we have witnessed a shift in our understanding of pain processing in humans from the peripheral nervous system to the central nervous system (CNS). Concurrent developments in methodologies that allow us to “image” brain activation in response to neuronal firing have enabled us to make this shift; we can readily and noninvasively determine the neuroanatomic substrate of pain perception in humans. Objectifying this subjective phenomenon has been the goal of pain researchers for decades, for obvious reasons. Slapping the cry baby at school and doing the same to a Tibetan monk would leave one sobbing but the other not so much as blinking. But is one really “feeling more pain” than the other at a physiologic level, or is it more a question of tolerance levels, coping, and attention, as well as individual past and present circumstances? The extent of tissue damage (or nociception) does not necessarily correlate with the amount of pain experienced for either of the subjects in the example, and neither do the behavioral changes measured (volume of vocalization, grimacing, limping, and so on). This nonlinear relationship between nociception and pain is not a novel concept; however, it does confound our ability to diagnose, prioritize, and treat pain patients. Pain is a conscious experience, an interpretation of the nociceptive input influenced by memories, and emotional, attentional, and cognitive factors. Pain is therefore a subjective experience. The International Association for the Study of Pain (1994) uses the following to define pain (53): “An unpleasant sensory and emotional experience associated with actual or potential tissue damage, or described in terms of such damage.”
This definition encapsulates the problem for researchers, physicians, therapists, care workers, and families who deal with pain patients. Pain is more than simple tissue damage and the brain has a central role to play in generating each individual’s pain experience. Clearly it would be useful to measure the levels of pain in patients objectively and reliably. It is not yet clear whether brain imaging methods provide an unequivocal level of objectivity. However, recent evidence supports their increasing utility in not only providing a noninvasive “assay” of nociception and, subsequently, pain perception, but also information about how central influences, such as sensitization/plasticity, attention, distraction, anxiety, depression, and other cognitive changes can impact the incoming nociceptive drive to produce an altered pain perception. Here, we review the field of pain imaging from the somatic and visceral pain fields and give specific examples of how these methodologies have contributed to our understanding of pain perception, its measurement and its modulation via behavioral and pharmacologic interventions.
BRAIN IMAGING AND THE NEUROANATOMY OF HUMAN PAIN PROCESSING
Functional brain imaging methods have revolutionized our understanding of how the human brain works, exploding onto the fields of neuroscience and medicine giving us unprecedented opportunities to “see” the brain in action (38,48,88). Pain research has benefited enormously from these developments; however, to better understand this literature it is useful to consider what aspects of brain activity we are measuring. Figure 13-1A displays the physiologic correlates of brain electrical activity. There is considerable evidence that local cerebral blood flow (CBF) changes reflect variations in local synaptic activity, as measured using positron emission tomography (PET) (75). The blood oxygenation level-dependent (BOLD) signal, detected using functional magnetic resonance imaging (fMRI) reflects simultaneously changes in local CBF and variations in deoxyhemoglobin (72). More recently, the neurophysiologic basis of the BOLD response has been further investigated and the findings confirm that the BOLD contrast mechanism reflects the input and intracortical processing of a
given area (44). Figure 13-1B displays how three other factors interact across these common brain imaging methods. In essence, you have a trade off between invasiveness and spatial/temporal resolution. It is clear from Figure 13-1B that fMRI scores reasonably well both spatially and temporally, and in addition is completely noninvasive. The noninvasive aspect of fMRI enables longitudinal studies to be performed safely, where patients can be followed and imaged several times during the course of their disease progression or therapeutic intervention. fMRI thus allows a broad range of sophisticated cognitive and neurophysiologic experiments to be performed, expanding our knowledge of brain function enormously and extending the early PET literature. A review of this material along with the pros and cons of PET versus fMRI is beyond the scope of this chapter; however, there are several excellent reviews and books that cover the basic principles, methods, and scientific contributions that fMRI and PET have made to neuroscience (24,38,48,49,63,77,88).
given area (44). Figure 13-1B displays how three other factors interact across these common brain imaging methods. In essence, you have a trade off between invasiveness and spatial/temporal resolution. It is clear from Figure 13-1B that fMRI scores reasonably well both spatially and temporally, and in addition is completely noninvasive. The noninvasive aspect of fMRI enables longitudinal studies to be performed safely, where patients can be followed and imaged several times during the course of their disease progression or therapeutic intervention. fMRI thus allows a broad range of sophisticated cognitive and neurophysiologic experiments to be performed, expanding our knowledge of brain function enormously and extending the early PET literature. A review of this material along with the pros and cons of PET versus fMRI is beyond the scope of this chapter; however, there are several excellent reviews and books that cover the basic principles, methods, and scientific contributions that fMRI and PET have made to neuroscience (24,38,48,49,63,77,88).
Most modern medical textbooks still claim that nociceptive signals arrive from the periphery via the spinal cord to the thalamus where they are distributed to sensory cortices, which process these signals to generate the conscious perception of pain. If pain imaging has contributed anything to the literature it is to dispel this myth. Summarizing the many excellent meta-analyses and reviews of the pain imaging literature that now exist (9,14,30,41,58,63), we can produce a more realistic diagram that highlights the key brain regions involved in processing nociceptive inputs to generate the conscious perception of pain in humans. This “pain matrix,” as it is often described, is shown in Figure 13-2. It should be remembered that the list of cortical regions is not extensive and how these regions interconnect to relay information and influence processing in specific brain regions is still being determined. The key to successful pain imaging is to dissect the multidimen-sionality of pain into dissociable brain regions that can then be targets for therapeutic intervention.
Varying combinations of factors (nerve damage, inflammation, hyperalgesia, anticipation, anxiety, fear, depression, etc.) within patients obviously lead to observable differences in clinical pain profiles, abilities to cope, and possibly the differences seen in treatment outcomes. Brain imaging can help us to “see” and thereby determine the extent to which each of these factors contribute to the patient’s pain providing useful information to guide diagnoses and appropriate treatment.
Albe-Fessard et al. (1) and Melzack (51) coined the idea that a neuromatrix for pain processing exists that comprises at least two main human nociceptive systems working in parallel: the medial and lateral pain systems. For many years a division of function within these pathways was asserted in that the lateral pathway processed the sensory-discriminatory aspects of pain experience and included structures such as the ventroposteriorlateral nucleus of the thalamus and sensory cortices, whereas the medial pathway processed the affective-cognitive-motivational aspects of pain processing and included structures such as the ventrocaudal part of the medial dorsal nucleus, anterior cingulate cortex, amygdala, and other limbic structures. It would appear, however, from various pain imaging experiments on both normal controls and increasingly in patients that this simple division of function between these parallel systems is not adequate
to explain all our findings. In a review, Price (67) puts forward a schematic, largely based on pain imaging data, that attempts to label specific brain regions with specific aspects of the pain experience from an acute nociceptive event toward the development of chronicity, during which the affective dimension becomes dominant. In this scenario, a nociceptive input first drives arousal, autonomic, and somatomotor responses that largely recruit the reticular formation of the brainstem, hypothalamus, supplementary motor area, and amygdala, but in addition there is an awareness of nociceptive sensations processed by the sensory cortices, posterior parietal complex, and insula cortex. Subsequent to this nociceptive sensation there is an assessment of perceived intrusion or threat that is most likely processed by the posterior parietal complex and insula cortex but influenced by the arousal and autonomic responses. There is then an immediate pain unpleasantness that is likely generated by the anterior cingulate cortex followed by second-order appraisals processed within prefrontal cortex with secondary pain affects manifest as the situation becomes chronic. This is an appealing framework that nicely fits with many imaging experiments; however, it does not take into account those situations where there is a top-down dysfunction that either generates or exacerbates pain perception. An alternative proposal for viewing pain has recently been proposed by Craig (12) that encourages us to think about pain as a homeostatic emotion rather than pain and temperature being an aspect of touch. Only time and further pain imaging experiments will confirm or refute this new concept for understanding pain processing, but it highlights how recent imaging data combined with conventional neurophysiologic measurements can provoke new ideas and hypotheses.
to explain all our findings. In a review, Price (67) puts forward a schematic, largely based on pain imaging data, that attempts to label specific brain regions with specific aspects of the pain experience from an acute nociceptive event toward the development of chronicity, during which the affective dimension becomes dominant. In this scenario, a nociceptive input first drives arousal, autonomic, and somatomotor responses that largely recruit the reticular formation of the brainstem, hypothalamus, supplementary motor area, and amygdala, but in addition there is an awareness of nociceptive sensations processed by the sensory cortices, posterior parietal complex, and insula cortex. Subsequent to this nociceptive sensation there is an assessment of perceived intrusion or threat that is most likely processed by the posterior parietal complex and insula cortex but influenced by the arousal and autonomic responses. There is then an immediate pain unpleasantness that is likely generated by the anterior cingulate cortex followed by second-order appraisals processed within prefrontal cortex with secondary pain affects manifest as the situation becomes chronic. This is an appealing framework that nicely fits with many imaging experiments; however, it does not take into account those situations where there is a top-down dysfunction that either generates or exacerbates pain perception. An alternative proposal for viewing pain has recently been proposed by Craig (12) that encourages us to think about pain as a homeostatic emotion rather than pain and temperature being an aspect of touch. Only time and further pain imaging experiments will confirm or refute this new concept for understanding pain processing, but it highlights how recent imaging data combined with conventional neurophysiologic measurements can provoke new ideas and hypotheses.
EXPERIMENTAL EVIDENCE
How reliable a “marker” of the subjective pain experience is the brain signal as measured by PET or fMRI? Confidence in the technique as an objective readout of the pain experience is only possible if this is true.
Coghill and colleagues (11) performed a study that examined pain intensity processing within the human brain using PET. In their study, they combined psychophysical assessment of graded nociceptive stimuli with PET to identify a brain network that perhaps subserved the processing of one dimension of the pain experience, namely, pain intensity. Multiple regression analysis revealed statistically reliable relationships between perceived pain intensity and activation of a functionally diverse group of brain regions, including those known to be important in sensation, motor control, affect, and attention. Pain intensity-related activation occurred bilaterally in the cerebellum, putamen, thalamus, insula, anterior cingulate cortex, and secondary somatosensory cortex, contralaterally in the primary somatosensory cortex and supplementary motor area, and ipsilaterally in the ventral premotor area. The authors conclude that their results confirm the existence of a highly distributed, bilateral brain mechanism engaged in the processing of pain intensity. They also conclude that the
conservation of pain intensity information across multiple, functionally distinct brain areas contrasts with the traditional view that sensory-discriminative processing of pain is confined within the somatosensory cortex, and perhaps accounts for the preservation of conscious awareness of pain intensity after extensive cerebral cortical lesions. Similar parametric studies have been done by others (7,18), which support the notion that brain activation, as detected with imaging methods, is a reliable readout of the subjective perception. Coghill et al. recently expanded this concept to address the issue that some individuals claim to be “sensitive” to pain, whereas others claim they tolerate pain well (10). Because it is difficult to determine whether these subjective reports reflect true interindividual differences in the experience, Coghill et al. combined psychophysical ratings to define pain report and “sensitivity” with fMRI to assess brain activity in 17 normal, healthy subjects. They found that highly “sensitive” individuals exhibited more frequent and more robust pain-induced activation of the primary somatosensory cortex, anterior cingulate cortex, and prefrontal cortex than did less “sensitive” individuals. For normal healthy controls, this study validates the subjective report as a reliable indicator of what is going on within the brain. However, in patients this might not be the case and divergence might exist between the report and the brain activation pattern because of the complexity of other factors that might contribute.
conservation of pain intensity information across multiple, functionally distinct brain areas contrasts with the traditional view that sensory-discriminative processing of pain is confined within the somatosensory cortex, and perhaps accounts for the preservation of conscious awareness of pain intensity after extensive cerebral cortical lesions. Similar parametric studies have been done by others (7,18), which support the notion that brain activation, as detected with imaging methods, is a reliable readout of the subjective perception. Coghill et al. recently expanded this concept to address the issue that some individuals claim to be “sensitive” to pain, whereas others claim they tolerate pain well (10). Because it is difficult to determine whether these subjective reports reflect true interindividual differences in the experience, Coghill et al. combined psychophysical ratings to define pain report and “sensitivity” with fMRI to assess brain activity in 17 normal, healthy subjects. They found that highly “sensitive” individuals exhibited more frequent and more robust pain-induced activation of the primary somatosensory cortex, anterior cingulate cortex, and prefrontal cortex than did less “sensitive” individuals. For normal healthy controls, this study validates the subjective report as a reliable indicator of what is going on within the brain. However, in patients this might not be the case and divergence might exist between the report and the brain activation pattern because of the complexity of other factors that might contribute.
The studies described teach us primarily about pain intensity; however, to disentangle components such as anticipation, fear, anxiety, attention/hypervigilance, or even depression first requires the development of novel cognitive and pharmacologic paradigms, initially applied to normal subjects in an experimental pain imaging laboratory. In our laboratory we have performed several such studies, some of which are discussed later (2,3,36,37,45,64, 65, 66,71,74,78, 79, 80, 81, 82, 83,91,92). More recent work has focused on examining the neural correlate of gender differences in pain perception and pain coping with the use of brain imaging (19,54). Differences have been observed both in terms of pain report and functional brain activation in several different brain regions, but a clear account of what regions are consistently differently activated and why requires further studies and investigation.
Other data from the laboratory of Borsook et al., and relevant to the field of headaches, has focused on determining the somatotopic activation in the human trigeminal pain pathways. They used fMRI to image pain-associated activity in three levels of the neuraxis: the medullary dorsal horn, thalamus, and primary somatosensory cortex by applying noxious thermal stimuli to facial skin at sites within the three divisions of the trigeminal nerve (V1, V2, and V3). Significant activation was observed in the ipsilateral spinal trigeminal nucleus within the medulla and lower pons in response to at least one of the three facial stimuli. In addition, activation from the three facial stimulation sites exhibited a somatotopic organization along the longitudinal (rostrocaudal) axis of the brainstem that was consistent with the classically described “onion skin” pattern of sensory deficits observed in patients after trigeminal tractotomy. Activation in the primary somatosensory cortex displayed a laminar sequence that resembled the trigeminal nucleus, with V2 more rostral, V1 caudal, and V3 medial. These findings were developed in a further study where the same authors determined the specific and somatotopic fMRI activation in the trigeminal ganglion by brush and noxious heat stimulation of the face within the receptive fields of each of the three divisions of the trigeminal nerve in controls. For both stimulus types, activation was somatotopically organized within the ipsilateral ganglion, as predicted by the known anatomic segregation of the neurons comprising the ophthalmic (V1), maxillary (V2), and mandibular (V3) divisions of the nerve. Signal decreased after brush stimuli and increased after the application of noxious heat. The abilities to detect somatotopic activation within the ganglion and to segregate non-noxious mechanical from noxious thermal stimuli suggest that fMRI will be valuable for measuring changes in the trigeminal ganglion in various clinical pain conditions (6,13).
Modulation of the Pain Experience
Descending Systems
Just as pain signals are important for survival, it is as important to regulate pain signaling in the nervous system. Head and Holmes postulated very early the existence of a descending pain modulatory system (28). Later this postulation was empirically confirmed (27) and provided a theoretical framework with the gate-control theory of Melzack and Wall (52). Wall also demonstrated a tonic regulatory influence from the brainstem on the spinal cord dorsal root level (87). The concept of the descending analgesic system was further developed when Mayer and Price demonstrated that stimulation in the periaqueductal gray matter produced analgesia without any concurrent effects on alertness or motor performance, so-called stimulusproduced analgesia (47). In the periaqueductal grey (PAG), ascending pain stimuli are integrated with descending influences from the diencephalon and the limbic forebrain. Important regions are the hypothalamus, the amygdala, the rostral components of the anterior cingulate cortex, insula, and the orbitofrontal cortex. PAG also receives influence from nearby nuclei of the catecholaminergic tone setting systems. Interestingly, microinjections of opioids into the amygdala produce analgesia, and analgesia that can be blocked by interference locally in the PAG (29). The PAG has strong bidirectional connections to the rostral medulla and this could be viewed as part of the pain modulation process given the role of the medulla in autonomic control. There are also strong suggestions that the analgesic
system is heavily related to the endogenous opioid systems (96).
system is heavily related to the endogenous opioid systems (96).
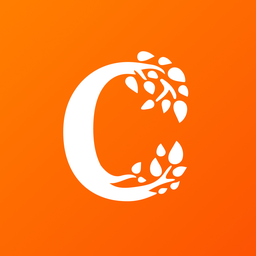
Full access? Get Clinical Tree
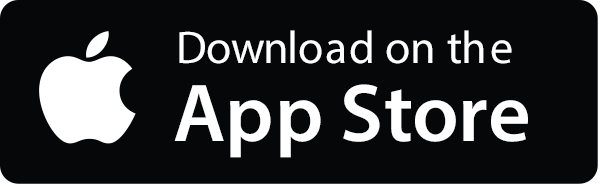
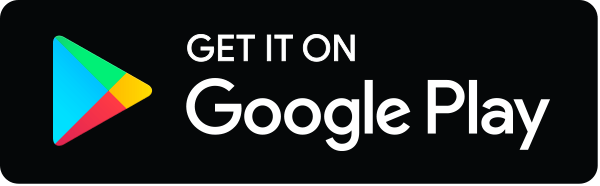
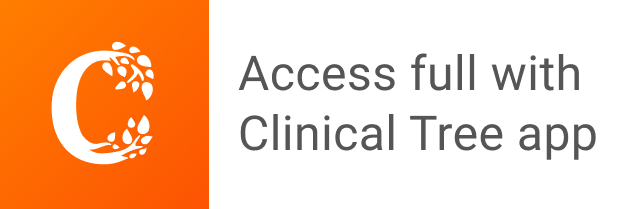