FIGURE 47.1 Final common pathway of shock. Hemorrhagic shock results in acute changes in circulating blood volume that culminates in a final common pathway shared by all classifications of shock.
Critical oxygen delivery is a function of cellular needs for oxygen and the ability of cells to extract oxygen from the arterial blood. Many factors contribute to this equation. During hemorrhage, tissue oxygen needs may increase due to increased respiratory muscle activity and increased catecholamine circulation (16). However, some evidence suggests that catecholamines downregulate the metabolic needs of cells during hypovolemic shock (2,3,17,18). Regional blood flow is modified during hypovolemic shock in an attempt to maintain oxygen delivery to critical tissues (14,19). In addition, the individual needs of various tissues may vary during hemorrhagic shock. For instance, the oxygen needs of the kidney may decline during hemorrhage because a fall in renal perfusion leads to a fall in glomerular filtration and a decrease in energy-consuming tubular absorption (14). In contrast, the gut may experience an increased oxygen debt early due to the high oxygen need of the mucosa, along with redistribution of blood away from the gut to more critical tissues. This is the physiologic basis for gastric tonometry as a means of measuring the adequacy of resuscitation early following hemorrhage (20).
Oxygen extraction in tissues is influenced by the position of the oxyhemoglobin dissociation curve (21–23). Factors that improve the ability of tissues to extract oxygen from hemoglobin (i.e., shift the curve to the right) include acidosis, hypercarbia, hyperthermia, and decreased blood viscosity. However, in any extreme, each of these factors can be overcome by inadequate oxygen delivery and cardiovascular collapse. Interestingly, the oxyhemoglobin curve has been shown to shift to the left in critically ill patients (24). The presence of 2,3-diphosphoglycerate (DPG) in transfused blood has also been associated with a left shift of the oxyhemoglobin dissociation curve (25). Thus, although transfusions may increase the hemoglobin level, theoretically improving oxygen delivery, they may negatively affect the ability of tissues to extract oxygen from the hemoglobin.
The severity of oxygen debt during hypovolemic shock has been shown to be a major determinant of survival in animals and in patients following trauma, hemorrhage, and major surgery (10,15,26,27). A large oxygen debt has been associated with the development of acute respiratory distress syndrome (ARDS) and multiple-organ dysfunction syndrome (MODS) (26,28–30). Conversely, a high oxygen delivery and uptake during resuscitation has been associated with improved survival (15,27,31–33). Whether increasing oxygen delivery to supranormal levels ultimately improves survival during resuscitation in critical illness remains controversial, and the medical literature has produced mixed results (28,34–37) with recent studies suggesting no clear benefit from this approach and concern that overly aggressive fluid resuscitation can lead to abdominal compartment syndrome and other complications (37).

FIGURE 47.2 Cellular mechanisms during anaerobic and aerobic glycolysis. In anaerobic conditions, pyruvic acid cannot enter the citric acid cycle within the mitochondria and is instead shunted to the production of lactate. This process produces only two molecules of ATP, as opposed to the 36 molecules of ATP produced from glucose in the mitochondria during aerobic glycolysis. Hydrolysis of ATP molecules in anaerobic conditions results in the production of hydrogen ions that cannot be cleared, leading to intracellular acidosis. (Adapted from Mizock BA, Falk JL. Lactic acidosis in critical illness. Crit Care Med. 1992;20:80–93.)
Cellular Response
During hypovolemic shock, the oxygen deficit in the tissues causes a fall in the mitochondrial production and concentration of high-energy phosphates because of greater breakdown than production (38–43). This led many researchers to evaluate the utility of adenosine triphosphate (ATP) in the resuscitation of hemorrhagic shock (44,45). In the presence of sufficient oxygen, aerobic combustion of 1 mol of glucose yields 38 mol of energy-rich ATP. However, in the absence of sufficient oxygen, glucose taken up by the cells cannot be combusted because of insufficient uptake of pyruvate into the mitochondrial tricarboxylic acid cycle. Pyruvate is then converted to lactate within the cytoplasm. Anaerobic glycolysis yields only 2 mol of ATP, which is then hydrolyzed into hydrogen ion, ultimately leading to intracellular and extracellular metabolic acidosis (Fig. 47.2) (38,39,46,47). This process is ultimately a function of the severity and duration of regional hypoperfusion relative to oxygen demand and is more pronounced in some tissues (diaphragm, liver, kidney, gut) than in others (heart, skeletal muscle). Ultimately, a significant fall in the high-energy phosphates for a prolonged duration will lead to irreversible cellular injury and death.
The sequelae of low ATP production are profound. About 60% of the energy produced by respiring cellular mitochondria is needed to fuel the sodium–potassium (Na+–K+) pump of the cell. This pump controls the gradient in electrolyte concentrations and electric potential over the cell membrane. In the absence of sufficient ATP, the Na+–K+ pump is inhibited, resulting in an influx of sodium into the cell and efflux of potassium out of the cell. This in turn leads to cellular fluid uptake (38,40,48–51). Hyperkalemia may result due to potassium exchange between cells, the interstitial fluid, and vascular space.
Independent of the Na+–K+ pump, there may be a selective increase in cell membrane permeability for ions during hemorrhagic shock. Hypovolemic shock has been shown to lead to a rapid decrease in the transmembrane potential (with a less negative inner membrane potential), resulting in rapid electrolyte and fluid shifts across the membrane. Circulating heat shock proteins may also contribute to these changes independent of energy deficit (51–54).
Finally, calcium (Ca2+) influx into cells and their mitochondria inhibits cellular respiration and ultimately contributes to cellular damage and swelling. Plasma levels of free Ca2+ may also fall. This may have profound consequences on the function of several organs during shock including the liver, kidney, heart, and vascular smooth muscle (49,50,55–61). Intracellular lysosomes lose their integrity, and proteolytic enzymes are released and contribute to cellular dysfunction and cell death. The sum of the intracellular changes and alterations in signaling transduction pathways described above ultimately leads to the development of cellular dysfunction and MODS, which may be irreversible (61).
Neurohumoral Response
In response to hemorrhage and hypovolemia, a complex neurohumoral response is initiated in an attempt to maintain blood pressure and retain fluid. Decreased intravascular volume stimulates baroreceptors in the carotid body and aortic arch, along with mechanoreceptors in the right atrium. This stimulation leads to several neurohumoral responses (Fig. 47.3). Circulating catecholamines are liberated by activation of the sympathetic nervous system and the adrenal medulla. Direct sympathetic stimulation of the vessel wall leads to vasoconstriction. Angiotensin II is liberated via the renin–angiotensin–aldosterone system. Vasopressin (antidiuretic hormone [ADH]) is released by the pituitary in hypovolemic shock and leads to vasoconstriction. Finally, decreased cardiac filling pressures reduce cardiac secretion of α-atrial natriuretic peptide (ANP), thereby reducing the vasodilatory and diuretic effects of ANP.
Macrocirculation
During loss of circulating blood volume, mechanisms are initiated to counteract the fall in cardiac output and oxygen delivery by facilitating a redistribution of peripheral blood flow (14). Regional autoregulation takes place via a delicate balance of endogenous vasodilators and vasoconstrictors. Endothelial cells produce potent vasodilators such as endothelium-derived relaxing factor (nitric oxide [NO]), heme oxygenation–derived carbon monoxide (CO), and metabolic byproducts in tissues, including carbon dioxide (CO2), potassium, and adenosine (62–69). Some authors describe that inhibition of NO early following hemorrhage ameliorates early hypotension and improves mortality (70–74). Conversely, other authors describe endothelial dysfunction in organs with diminished NO production (75,76). Endothelin is a potent endothelial cell–derived vasoconstrictor that is released upon catecholamine stimulation or hypoxia (77). The overall increase in systemic peripheral vascular resistance is distributed differently among various organs in the body (19). Vasoconstriction also occurs in the venous vasculature, increasing return of available blood to the heart (4,78). The complex interplay of these mechanisms for vasodilation and vasoconstriction ultimately determines the regional redistribution of blood flow to organs following hemorrhagic shock. The redistribution of blood flow results in a greater share of oxygen delivery to organs with high obligatory metabolic demands (heart and brain), and a lesser share to those with fewer demands including the skin, skeletal muscle, kidney, intestine, and pancreas (3,19,66,79).

FIGURE 47.3 Neurohormonal response to hemorrhage. Hemorrhage results in a decrease in the circulating intravascular volume, which initiates a complex cascade of compensatory events. CNS, central nervous system; ACTH, adrenocorticotropic hormone; ADH, antidiuretic hormone.
Microcirculation
One of the most important determinants of tissue perfusion during shock is the response and function of the microvasculature, which is defined as vessels less than 100 to 150 μm in diameter. Although arteries and medium-sized arterioles constrict in response to the extrinsic control mechanisms described above, terminal arterioles, venules, and capillaries remain unaffected and are more controlled by local metabolic factors.
Alterations in microvascular function and flow are affected through precapillary and postcapillary sphincters, which are sensitive to both extrinsic and intrinsic control mechanisms. Exchange of metabolites and compartmental regulation of fluids occur at the capillary level. Therefore, alteration of tone of the pre- and postcapillary sphincters can have significant effects on microcirculatory function (78,80,81). Failure to dilate sphincters supplying metabolically active tissues may result in ischemia and anaerobic metabolism with lactate production. Increased precapillary tone, as seen with sympathetic stimulation, results in increased blood pressure systemically and decreased hydrostatic pressure locally. In fact, the microvascular arterioles may even dilate in response to the above vasoconstriction due to release of metabolic byproducts of underperfusion (carbon dioxide, hydrogen ion, etc.). The decrease in hydrostatic pressure locally then leads to redistribution of fluid from the interstitium to the circulation. Conversely, increased postcapillary tone (relative to precapillary tone) results in vascular pooling of blood and loss of fluid to the interstitium (as a result of increased hydrostatic pressure). This increased hydrostatic pressure may become accentuated in response to crystalloid resuscitation, leading to interstitial edema (81). Finally, hemorrhage and shock have also been shown to induce increased permeability of capillaries, leading to interstitial fluid leak during resuscitation (82,83).
Hypovolemic shock and hemorrhage also induce the expression of endothelial adhesion molecules on neutrophils and endothelium (48,84). This results in neutrophil adherence and “rolling” of cells within the capillary bed (85–87). Capillary flow then diminishes and may also impair red blood cell flow. While this decrease in transit time may augment the ability of tissue to extract oxygen, it may also lead to microvascular thrombosis and further tissue ischemia (88,89).
Metabolic and Hormonal Response
The early hyperglycemic response to trauma/hemorrhage is the combined result of enhanced glycogenolysis, caused by the hormonal response to stress including elevated epinephrine, cortisol, and glucagon levels; increased gluconeogenesis in the liver, partly mediated by glucagon; and peripheral resistance to the action of insulin (38,90). Increased gluconeogenesis in the liver, and to a lesser extent in the kidneys, follows increased efflux of amino acids, such as alanine and glutamine from the muscle to the liver, due to a breakdown of muscle protein. The latter is evidenced by increased urinary losses of nitrogen and a negative nitrogen balance. Lactate produced in muscle can also be converted to glucose in the liver (91). Increased epinephrine also results in skeletal muscle insulin resistance, sparing glucose for use by glucose-dependent organs such as the heart and brain. Later in shock, hypoglycemia may ensue, possibly because of glycogen depletion or hepatic ischemia (38,91,92). Fatty acids are increased early in shock, but later levels fall (90). Without energy for glycolysis, the cell depends on lipolysis and the autodigestion of intracellular protein for energy. Initially, ketone bodies and the branched-chain amino acids are used as alternative fuel sources. Without oxygen, these sources become inefficient, leading to hypertriglyceridemia, increased β-hydroxybutyric acid and acetoacetate levels, and changes in the amino acid concentration pattern. As these metabolic changes occur, set in motion by cellular hypoxia and promoted by systemic hormonal changes, structural changes occur within individual cells (93).
Inflammatory and Immune Response
A detailed discussion of the inflammatory and immune response to trauma and hemorrhage is beyond the scope of this chapter. However, several general concepts can be introduced. Following hemorrhage and resuscitation, macrophages, including lung macrophages and Kupffer cells in the liver, may release pro-inflammatory cytokines such as tumor necrosis factor (TNF)-α and interleukin (IL)-1, -6, and -8. During reperfusion, cytokines may induce and amplify the inflammatory response to ischemia and may further induce local and remote organ damage (94–104). The reperfused gut, for example, may, together with the liver, be a source of systemically circulating cytokines, and possibly endotoxin. Release of mediators into the mesenteric lymph, portal, or systemic circulations during reperfusion may have deleterious effects on remote organs, such as the lungs, due to neutrophil activation and adherence, leading to pulmonary vascular injury with increased permeability (95,98,99). Antigen–antibody complexes activate the complement cascade, and complement fragments thus generated can interact with other cytokines to promulgate the inflammatory response. Complement activation can yield potent vasodilating and leuko-attractant substances (105–108).
Oxygen radicals, such as hydrogen peroxide and superoxide anion, are released by activated neutrophils in response to a variety of stimuli. They are also released when xanthine oxidase is activated after reperfusion in ischemia–reperfusion models. These highly reactive products lead to cell membrane dysfunction, increased vascular permeability, and release of eicosanoids (109–113).
This inflammatory process results in the local accumulation of activated inflammatory cells, which release various local toxins such as oxygen radicals, proteases, eicosanoids, platelet-activating factor, and other substances. When unregulated, such accumulations can cause tissue injury. The initial attachment of neutrophils to the vascular endothelium at an inflammatory site is facilitated by the interaction of adherence molecules on the neutrophil and endothelial cell surfaces (114–119).
Trauma-Induced Coagulopathy
Patients suffering from severe hemorrhagic shock will frequently develop a coagulopathy that can further complicate the control of hemorrhage. Previously, this coagulopathy was thought to be primarily due to loss of clotting factors during hemorrhage combined with dilution during the resuscitation phase. Recent evidence suggests that 25% to 40% of severely injured patients are already manifesting evidence of coagulopathy at the time of hospital admission (120,121). This has been termed trauma-induced coagulopathy. The etiology of trauma-induced coagulopathy is likely multifactorial and six key initiators of coagulopathy have been described (Fig. 47.4). These include tissue trauma, shock, hypothermia, academia, hemodilution, and inflammation (122). Tissue injury leads to exposure of subendothelial type III collagen and tissue factor, which binds von Willebrand factor and platelets inducing coagulation. Hyperfibrinolysis also results from tissue injury due to release of endothelial tissue plasminogen activator and inhibition of plasminogen activator inhibitor-1 in the setting of shock (122). Hypothermia and academia can also directly impair coagulation protease activity. Recent data also suggest that depletion of coagulation factors early after injury may be driven by the protein C system (121). Over time, trauma patients who are initially coagulopathic with increased bleeding will transition to a hypercoagulable state, which increases the risk of thrombotic complications. Additional work is regarded to better understand the timing and mechanism of this transition. Evidence of trauma-induced coagulopathy upon hospital admission has been associated with a fourfold increase in mortality (123,124). Therefore, acute measurement of coagulation parameters during resuscitation from hemorrhagic shock is indicated along with early efforts to address coagulation abnormalities.

FIGURE 47.4 Mechanisms of trauma-induced coagulopathy. Trauma results in hemorrhage, which leads to resuscitation, which in turn leads to dilution and hypothermia causing coagulopathy and further hemorrhage. This is the classic, “dilutional coagulopathy.” Hemorrhage also causes shock, which causes acidosis and hypothermia that also contribute to coagulopathy. Finally, trauma and shock can also cause acute coagulopathy of trauma–shock associated with factor consumption and fibrinolysis. Coagulopathy is further associated with trauma-induced inflammation and modified by genetics, medications and acquired diseases. (With permission from Hess JR, Brohi K, Dutton RP, et al. The coagulopathy of trauma: a review of mechanisms. J Trauma. 2008;65:748–754.)
DIAGNOSIS
Early diagnosis of hemorrhagic shock is imperative to avoid delay in treatment. However, clinical signs are relatively insensitive for small amounts of blood loss. There is a progressive hemodynamic deterioration with ongoing blood loss. This classic progression is delineated in Table 47.1. Total blood volume is estimated at approximately 70 mL/kg in the average adult, or nearly 5 L for a 70-kg person. The signs and symptoms of hemorrhagic shock vary based on the severity of blood loss. Traditionally, this progression has been defined as four classes of shock.
Class I hemorrhage is marked by a less than 750 mL estimated blood loss, or less than 15% of total circulating blood volume. There are minimal physical signs associated with this volume of blood loss. The patient may not have tachycardia, with a heart rate remaining less than 100 beats per minute; the systolic blood pressure and pulse pressure remain normal; the respiratory rate remains at 14 to 20 breaths per minute; and urine output remains adequate (>30 mL/hr). Only subtle physical signs such as delayed capillary refill and slight anxiety may exist.
Class II hemorrhage is marked by an estimated blood loss of 750 to 1,500 mL (or 15% to 30% of the total circulating blood volume). Physical signs begin to manifest during this stage of hemorrhage. Although the systolic blood pressure may be maintained, the patient usually becomes tachycardic (heart rate greater than 100 beats per minute), the pulse pressure begins to decrease, and capillary refill is delayed. The respiratory rate begins to increase (20 to 30 breaths per minute), urine output becomes diminished (20 to 30 mL/hr), and the patient becomes very anxious.
Class III hemorrhage is marked by an estimated blood loss of >1,500 to 2,000 mL (or >30% to 40% of total circulating blood volume). During this phase, significant hemodynamic compromise becomes apparent. Heart rate increases to >120 beats per minute, systolic blood pressure decreases, pulse pressure decreases, capillary refill decreases, tachypnea worsens with a respiratory rate of 30 to 40 breaths per minute, urine output drops to 5 to 15 mL/hr, and the patient becomes confused, showing further evidence of decreased perfusion of the central nervous system.
Class IV hemorrhage is marked by an estimated blood loss of >2,000 mL (or >40% of total circulating blood volume). During this phase, most compensatory cardiovascular mechanisms have been maximized and total hemodynamic collapse is imminent. Signs of class IV hemorrhage include severe tachycardia with a heart rate >140 beats per minute, a decreased systolic blood pressure, a decreased pulse pressure, delayed capillary refill, significant tachypnea with a respiratory rate of >35 breaths per minute, minimal to no urine output, and severely altered mental status as marked by confusion and/or lethargy.
Another way to classify patients with hemorrhagic shock is based on their response to initial fluid resuscitation. Rapid responders will typically require a limited fluid resuscitation to normalize vital signs. Transient responders will improve following fluid administration but then hypotension will recur. Nonresponders remain hypotensive despite fluid and blood product administration. Generally transient responders and nonresponders will require additional intervention to obtain hemorrhage control (Table 47.2).
TABLE 47.1 Clinical Classes of Hemorrhagic Shock |
![]() |
TABLE 47.2 Response to Initial Fluid Resuscitation and Patient Management |
![]() |
Transient responders can be the most difficult to diagnose. Retrospective studies have shown that patients with field hypotension who become normotensive on arrival to the emergency department have increased morbidity, mortality, need for operation, and admission rate to the intensive care unit (ICU) (125–127). Approximately 15% of these patients will need transfusion, with 37% requiring therapeutic surgery (127). Hence, even a brief episode of hypotension can be a marker for significant underlying injury. Tachycardia is one of the first signs of shock, although some patients may respond to traumatic hemorrhage with bradycardia as a result of a vagal nerve–mediated transient sympathoinhibition due to acute and sudden blood loss (1–3,128,129). Narrowing of the pulse pressure, low end-tidal CO2, and markers of acidosis (base deficit or lactate) are also useful predictors of compensated shock (130–134).
Special Populations
Geriatrics
Concurrent medication, such as β-blockers, may attenuate the physiologic response to hemorrhage. In the presence of β-blockade, tachycardia may be blunted or may not occur at all. Prior hydration status and use of diuretics can also alter the rate at which these signs present. Elderly patients may have atrial arrhythmias leading to a high ventricular response, making tachycardia less sensitive in this patient population Changes in blood pressure in geriatric patients may also be more difficult to assess. Those with a baseline hypertension may have what appears to be a normal adult blood pressure but may be in compensated shock and have significant organ hypoperfusion (130,135,136).
Pregnant Patients
Pregnant patients have a significantly increased total blood volume, and thus can lose up to 1,000 mL of blood before presenting with any clinical signs of hemorrhage. Blood is diverted from the placenta via vasoconstriction; the mother’s total blood circulation is maintained at the expense of the fetus. Fetal distress may be the first sign of shock in the mother (137,138).
Pediatrics
Pediatric patients have extensive compensatory mechanisms, which can result in significant tachycardia before any hypotension is manifested. Hypotension in a pediatric patient is an ominous sign and suggests advanced stages of hemorrhage.
Diagnostic Approach
The first priority in managing a patient with hemorrhagic shock is to identify and control the source of bleeding. If no obvious source of external bleeding is identified, a rapid evaluation should be performed to identify likely occult sources of bleeding. In the trauma patient, significant internal hemorrhage can occur in four defined regions: the thoracic cavity, the peritoneal cavity, the retroperitoneum, and extremity fractures. These areas can be rapidly assessed via chest radiograph, a pelvic radiograph, focused assessment with sonography for trauma (FAST), and physical examination of extremities along with appropriate radiographs. In-depth coverage of the diagnosis of abdominal trauma is provided in a later chapter of this book. In nontrauma patients without clear evidence of bleeding, the gastrointestinal tract should be rapidly evaluated via nasogastric tube, rectal examination, and endoscopy where appropriate. Additional diagnostic tests can be obtained based on clinical history, patient background, and condition. Abdominal aortic aneurysms can be identified on physical examination and bedside ultrasound. In selected instances, angiography may be used to identify and treat sources of hemorrhage not otherwise apparent (pelvic fractures, pancreatitis, lower gastrointestinal bleeding) (139–144). This should only be instituted when a specific source of hemorrhage is highly likely and therapeutic intervention is sought. Computed tomography should be avoided in hemodynamically unstable patients with hemorrhage.
Laboratory Testing
Hematocrit and Hemoglobin
Hemoglobin and hematocrit measurements have long been part of the basic diagnostic workup of patients with hemorrhage and/or trauma. However, in patients with rapid bleeding, a single hematocrit measurement on presentation to the emergency department may not reflect the degree of hemorrhage. In a short transport or presentation time, prior to initiation of resuscitation, the body’s compensatory mechanisms for fluid retention and resorption into the vascular space have not taken place, and initial hematocrit levels may remain stable despite significant blood loss. A retrospective study of 524 trauma patients (145) determined that the initial hematocrit had a sensitivity of only 0.50 for detecting patients with an extent of traumatic hemorrhage requiring surgery. The diagnostic value is further confounded by the administration of intravenous fluids and red cell concentrates during resuscitation (146–148).
Two prospective observational studies determined the sensitivity of serial hematocrit measurements for detecting patients with severe injury (148,149). In the first study (148), the authors compared values of hematocrit at admission and 15 and 30 minutes following arrival to the emergency department. A normal hematocrit on admission did not preclude significant injury. The mean change in hematocrit levels between arrival and 15 minutes, and 15 and 30 minutes was not significantly different in patients with or without serious injuries. However, a decrease of hematocrit by over 6.5% at 15 and 30 minutes had a high specificity for injury (0.93 to 1.0), but a low sensitivity (0.13 to 0.16).
Another prospective observational study examined the utility of serial hematocrit measurements during the initial 4 hours following admission (149). A significant limitation to this study is that they removed patients who required a blood transfusion in order to eliminate confounding variables. In the remaining 494 patients, a decrease in hematocrit of more than 10% between admission and 4 hours was highly specific for severe injury (0.92 to 0.96), but again, it was not sensitive (0.09 to 0.27).
Overall, decreasing hematocrit levels over time may reflect continued bleeding. However, patients with significant bleeding may maintain their hematocrit level, especially in the absence of resuscitation. Conversely, hematocrit levels may also be confounded by aggressive fluid resuscitation early during resuscitation (146,147). An initial hematocrit level will help to identify patients who present with pre-existing anemia who may have a lower threshold for hemorrhage. The hematocrit level should be used in conjunction with other measures of perfusion in order to determine the presence of occult hemorrhage.
Measurements of Perfusion
Lactate
Lactate was initially suggested as a diagnostic parameter and prognostic indicator of hemorrhagic shock in the 1960s. Substantial data exist that lactate levels as a marker of tissue oxygen debt can predict outcome in various forms of shock (46,150). Recent studies have suggested that early changes in lactate even in the prehospital environment may be predictive of the need for blood transfusion and hemorrhage control procedures. A recent multicenter trial which enrolled 327 trauma patients with a systolic blood pressure ≤100 mmHg, transported by ground advanced life-support services reported that prehospital lactate ≥2.5 mgmol/L was a better predictor than blood pressure or shock index for the need for resuscitative care (151).
During hemorrhage, not only is the initial lactate level important, but also the rate of clearance (152,153). Two prospective studies confirm this. In one prospective observational study (152), 76 patients with multiple trauma were analyzed with respect to clearance of lactate between survivors and nonsurvivors over 48 hours. If lactate normalized within 24 hours, survival was 100%. Survival decreased to 77.8% if normalization occurred within 48 hours, and to 13.6% in those in whom lactate levels remained elevated above 2 mEq/L for more than 48 hours. This was confirmed in another prospective study of 129 trauma patients (153) in which initial lactate levels were higher in nonsurvivors. A prolonged time to normalization (>24 hours) was associated with the development of posttraumatic organ failure. Finally, venous lactate has been shown to be an excellent approximation for arterial lactate in acute trauma patients and is a useful marker for significant injury (154).
Taken together, these studies suggest that both the initial lactate level and the rate of clearance are reliable indicators of morbidity and mortality following trauma. However, whether lactate should be used as an end point of resuscitation or is merely a marker of tissue ischemia has not been clearly established.
Base Deficit
Base deficit values derived from arterial blood gas analysis have also been shown to provide an indirect estimation of tissue acidosis due to impaired perfusion (155–161). However, base deficit can be affected by resuscitation fluids (hyperchloremic metabolic acidosis) and exogenous administration of sodium bicarbonate. Despite these potential drawbacks, initial base deficit has been shown in several retrospective studies to correlate with transfusion requirements, organ dysfunction, morbidity, and mortality following trauma (26,162–167). The magnitude and severity of the base deficit also correlates to outcome, and is useful in both pediatric and elderly patients (165,166). Base deficit has been shown to be a better predictor of outcome than pH alone following traumatic injury (164).
Lactate versus Base Deficit
Although many studies have shown that both base deficit and serum lactate levels correlate with outcome following trauma and hemorrhage, these two parameters do not always correlate with each other (168,169). In fact, lactate has been found to be a superior predictor of mortality as compared to base deficit in a recent study of patients in the ICU following trauma (168). Both base deficit and lactate have been shown to correlate to outcome in nontraumatic etiologies of hemorrhagic shock (170,171). Given that there are confounding variables following trauma that can affect measured levels of both lactate and base deficit, independent assessment of both parameters along with the patient’s clinical condition is recommended for the evaluation of shock in trauma patients.
Measurement of Coagulopathy
Standard Coagulation Studies
Traditional studies of coagulation include prothrombin time (PT), activated partial thromboplastin time (aPTT), fibrinogen level, and platelet count. Although no tightly controlled trials have been performed, current recommendations for therapeutic end points in hemorrhagic shock include maintaining PT and aPTT at less than 1.5 times the normal value, maintaining a platelet count of >100 in patients with active bleeding or traumatic brain injury, and maintaining a fibrinogen level of >1 g/L.
While these laboratory studies are standard, they do present several drawbacks. To begin, in vivo coagulation depends on the interaction between platelets and coagulation factor enzymes. Laboratory values of PT and aPTT are performed on platelet-poor plasma and fail to evaluate the cellular interactions of clotting. PT and aPTT measurements also do not take into account hypothermia-induced coagulopathy because samples are warmed prior to measurement. Platelet and fibrinogen assays give numerical values, but fail to assess function. Finally, each of these tests takes time, in most centers up to 30 to 45 minutes. This lag time makes these studies clinically inefficient because when the results become available, they may not truly reflect the patient’s clinical condition. During resuscitation, actively bleeding patients are in a constant state of flux. One study has suggested that with efficient laboratory processing and emergency hemorrhage panel which includes PT/INR, platelet count, and fibrinogen can have results available within 20 minutes (172). Alternative point-of-care testing such as the iSTAT handheld analyzer can provide rapid bedside results, but is currently limited to activated clotting time (ACT) and PT/international normalized ratio (INR) (173).
Thromboelastography
The thromboelastograph (TEG) analyzer is a bedside machine that provides a functional evaluation of overall coagulation on whole blood at the same temperature as the patient. The TEG has been shown to be a more sensitive measure of coagulation disorders than standard coagulation measures (174). The TEG assay provides a tracing that measures clotting (R value), clot formation (α angle), clot strength (maximum amplitude [MA]), and clot lysis (LY 30) (Fig. 47.5). Elongation of the R value represents a deficiency in coagulation factors. The α angle represents the rate of fibrin accumulation and cross-linking, which can be affected by fibrinogen function and, to a lesser degree, platelet function. The MA is a measure of clot strength and is affected primarily by platelets and, to a lesser degree, fibrinogen. A recent study investigating the utility of the admission TEG in trauma patients found that the TEG data were superior to conventional coagulation tests at predicting coagulopathy. The TEG data identified patients at increased risk for early transfusion and identified patients with fibrinolysis (175). Several authors have proposed TEG-guided resuscitation strategies for trauma patients with hemorrhagic shock (175,176).

FIGURE 47.5 Thromboelastogram. The thromboelastograph (TEG) analyzer is a bedside machine that provides a functional evaluation of overall coagulation on whole blood at the same temperature as the patient. The thromboelastograph assay provides a tracing that measures time to clot formation (R value), speed to a certain clot strength (K value), rate of clot formation (α angle), overall clot strength (maximum amplitude [MA]) and clot lysis (LY 30).
TREATMENT
Trauma is by far the most common etiology for hemorrhagic shock. While other causes do exist, management priorities are similar regardless of the source of bleeding. Diagnosis, evaluation, and management must often occur simultaneously. A methodical approach is necessary to optimize outcome (Table 47.3). Unique to hemorrhagic shock, as opposed to other forms of shock, is that definitive management frequently requires surgical or procedural intervention to cease bleeding. The diagnostic pathway and interventions pursued become part of the resuscitation pathway. What follows is a summary of the interventions, diagnostic studies, monitoring strategies, and resuscitation techniques for hemorrhagic shock.
Immediate Management
When approaching any patient in shock, the sequence of events should be to address the issues of airway, breathing, and circulation—also known as the “ABCs.” Most patients with fully developed shock require tracheal intubation and mechanical ventilation, even if acute respiratory failure has not yet developed. Studies have shown that during shock, the respiratory muscles require a disproportionate percent of the cardiac output (16). Failure to mount a hyperventilatory response to a metabolic acidosis is a significant predictor of the need for subsequent intubation in trauma patients (177). Mechanical ventilation allows flow to be redistributed, lessens the work of breathing, may help reverse lactic acidosis, and supports the patient’s airway until other therapeutic measures can be effective. Tracheal intubation is also required if there is evidence of mental status changes, such that airway protection is questionable. Evidence of hypoxemia and/or hypoventilation is also an absolute requirement for early intubation.
TABLE 47.3 Key Steps in the Approach to a Patient with Hemorrhagic Shock |
![]() |
Perhaps most complex is the patient with evidence of compensated hemorrhagic shock whose mental status is still intact. In this type of patient, clinical acumen is imperative. If the initial response to resuscitation is sustained (i.e., “a responder”), then close observation of the airway may be appropriate while additional workup and treatment are pursued. However, in a patient who is not responsive or has a transient response to fluid resuscitation, control of the airway early is necessary prior to respiratory collapse (177). In addition, if diagnostic and therapeutic interventions, such as angiography and embolization, are required during resuscitation to control hemorrhage, early airway control should be obtained.
Once the airway is secured, it is important to closely monitor techniques of ventilation. Studies have shown that there is a tendency of rescue and medical personnel to hyperventilate patients during resuscitation (178,179). Hyperventilated patients have been shown to have an increased mortality when compared to nonhyperventilated patients in the setting of severe traumatic brain injury (180–182). Animal studies have supported this information, showing that cardiac output increases with hypoventilation and decreases with hyperventilation and positive end-expiratory pressure (PEEP) (183,184). Thus, adequate appropriate ventilator strategies are imperative early in hemorrhagic shock to optimize tissue perfusion and outcome.
The management steps to restore adequate circulation include identifying and controlling the source of hemorrhage and intravenous fluid and blood product resuscitation to restore tissue perfusion. The first step is to control obvious hemorrhage immediately.
Hemorrhage Control
Resuscitation of the bleeding patient requires early identification of potential bleeding sources followed by prompt action to minimize blood loss, restore tissue perfusion, and achieve hemodynamic stability. This is particularly important in the trauma patient where multiple sources may be involved. Wound compression is the initial maneuver to control an exsanguinating wound. For massive soft tissue injuries or major vascular injury to an extremity, placing a tourniquet proximally may decrease hemorrhage and allow resuscitation prior to definitive control (185). Fractures should be splinted or placed in traction. Evidence of pelvic instability or hemorrhage may be temporized by a sheet or a pelvic binder (186,187). In the presence of massive trauma, patients may present with coagulopathy in the emergency department and this should be preemptively addressed. The same principles should be applied to nontraumatic hemorrhagic shock, such as gastrointestinal bleeding and ruptured aortic aneurysms: rapidly identify and attenuate the obvious sources of hemorrhage.
Multiple studies have confirmed that patients in need of emergency surgery for ongoing hemorrhage have a better survival if the elapsed time to definitive care is minimized (188–192). Those patients with unnecessary delays in diagnosis and definitive treatment will have increased morbidity and mortality. A multicenter retrospective review of over 500 deaths in the operating room concluded that delayed transfer to the operating room was a cause of death that could be avoided by shortening the time to diagnosis and resuscitation (193). The development of trauma systems has significantly contributed to improved trauma outcomes by triaging more severely injured patients to hospitals that have systems in place to rapidly diagnose, resuscitate, and definitively treat patients with hemorrhagic shock (188–190). The implementation of trauma systems has resulted in improved outcomes in severely injured patients, decreased time to operating room in hypotensive patients, decreased complications, decreased hospital length of stay, and decreased mortality, especially in patients with severe injury as measured by an ISS of >15 (190). Definitive prompt care is critical to optimize outcomes in patients with trauma and hemorrhage.
Intravenous Access
Access to the bloodstream should be obtained expediently. Two peripheral large-bore intravenous catheters (18 gauge or larger) are necessary. If cannulation of a peripheral vein is difficult due to collapse, then central venous access should be secured. In the presence of trauma to the torso, venous access above and below the diaphragm is preferable. When obtaining intravenous access, it is important to note that the maximal rate of infusion via a catheter is directly proportional to the diameter of the catheter and indirectly proportional to the length. Therefore, a 9 French percutaneous introducer sheath will infuse fluids more rapidly than a 7 French triple-lumen catheter. A large-bore peripheral intravenous catheter will also infuse fluids more rapidly than a 7 French triple lumen catheter due to a shorter length and less resistance. Intraosseus access is an option for both adults and children when i.v. access is difficult. Fluids and blood products can be delivered via this route. However, this should be considered temporary access until better intravenous access can be obtained.
Adjunctive Measures
Historical teachings have been that tilting a patient into head-down position (i.e., Trendelenburg) diverts blood volume into the central circulation and improves venous return, thereby improving stroke volume and cardiac output in hypovolemic shock. However, studies do not show any significant redistribution of blood volume centrally (194). In fact, the head-down position can worsen gas exchange and cardiac function. Therefore, the Trendelenburg position is no longer recommended as a resuscitative technique. If this type of measure is deemed desirable, raising the legs above the level of the heart should be adequate (195).
The use of pneumatic antishock garments (PASGs, previously military antishock trousers [MAST]) currently has a limited role in the management of hypotensive trauma patients. Although their use was almost universal for hemorrhage control in the late 1970s and 1980s, recent studies have demonstrated that they have no effect on patients with thoracic injury. In fact, some evidence suggests that mortality is higher when PASGs are applied (196,197). No survival advantage has been demonstrated in the pediatric population, although there may be a small survival benefit in children with a systolic blood pressure of less than 50 mmHg (198). The main utility of PASGs currently is as a temporizing agent to stabilize pelvic fractures.
Fluid Resuscitation
Careful attention to fluid resuscitation is necessary during management of hemorrhagic shock to optimize outcome. It is still unclear which type of fluid should be employed in the initial treatment of the bleeding patient.
Several meta-analyses have shown an increased risk of death in patients resuscitated with colloids as compared with crystalloids (199–202) during hemorrhagic shock. While three of these studies suggested that the effect was particularly significant in the trauma population, the results of a recent meta-analysis showed no significant difference (203). A trial evaluating 4% albumin versus 0.9% normal saline in nearly 7,000 ICU patients showed that albumin administration was not associated with worse outcome. There was a trend, however, toward higher mortality in the trauma subgroup that received albumin (p = 0.06) (204). The difficulty with interpreting these meta-analyses and the individual studies is that they are very heterogeneous. Each evaluates different patient populations and resuscitation strategies, and mortality may not always be a primary end point. However, given these results, crystalloid resuscitation is currently the accepted standard as initial therapy for hemorrhagic shock.
Many synthetic colloid solutions such as hetastarch and dextran have also been associated with coagulopathy. Recent research suggests that hetastarch solutions with a high mean molecular weight and a high C2/C6 ratio suppress coagulation more than solutions with rapidly degradable low–molecular-weight colloids (205–207). This coagulopathy may be produced by one of several potential mechanisms including a reduction in von Willebrand factor, platelet dysfunction, reduced factor VII levels, and an interaction with fibrinogen (173,208).
Crystalloid solutions are not without side effects. Resuscitation with fluids that contain supraphysiologic concentrations of chloride can lead to hyperchloremic acidosis. This can be significant in patients where lactic acidosis may already be present. Lactated Ringer solution contains a more physiologic concentration of chloride (109 mEq/L) than normal saline (NS 154 mEq/L), and therefore may be the preferred choice. Animal studies have also shown that resuscitation with normal saline can lead to more coagulopathy and increased blood loss than resuscitation with lactated Ringer solution (209).
Massive resuscitation with crystalloid fluids alone can lead to several significant complications including cardiac and pulmonary complications, gastrointestinal dysmotility, coagulation abnormalities, and immunologic dysfunction (210). Reports of lactated Ringer solution and normal saline increasing reperfusion injury and leukocyte adhesion suggest that crystalloid resuscitation may worsen acidosis and coagulopathy in severely injured patients and possibly increases the risk of ARDS, systemic inflammatory response syndrome (SIRS), and multi-organ failure (MOF) (210–213). Abdominal compartment syndrome has been clearly associated with excessive use of crystalloid resuscitation (214–218). Recently, there has been increased focus on early use of blood products in order to minimize crystalloid use in the resuscitation of hemorrhagic shock. Finally, resuscitation strategies that focus on early aggressive fluid resuscitation to normalize blood pressure before bleeding is controlled may result in increased hemorrhage and increased mortality. This has led some authors to suggest that “hypotensive resuscitation” should be the goal until the source of hemorrhage is controlled (219–222). However, the exact goals for mean arterial pressure and trigger points for bleeding have not been established. The potential adverse sequelae when used in patients with associated injuries or comorbidities (i.e., severe closed head injury) have not been clearly established.
TABLE 47.4 Damage Control Laparotomy and Damage Control Resuscitation |
![]() |
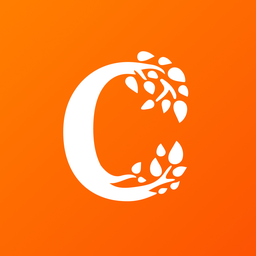
Full access? Get Clinical Tree
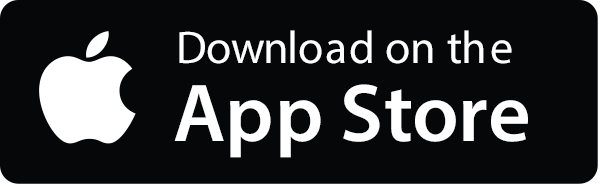
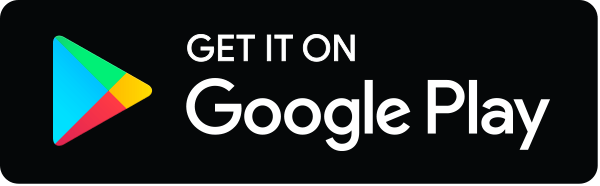