The negative intrathoracic pressure not only supports ventilation of the lung but also promotes central venous return, right atrial filling, and right ventricular preload.2–7 Augmentation of venous return occurs when the diaphragm contracts pressing down on the abdominal cavity. This downward movement compresses the mesenteric venous complex, thus promoting return of this blood volume to the central venous circulation. Known as the “thoracic pump,” this is one of two mechanisms supporting venous return to the right side of the heart. The second mechanism, the “skeletal pump,” promotes venous return through contraction of muscles around the peripheral veins (Figure 14-2).3,4,7,8 Contraction of the skeletal muscles compresses the veins, propelling venous blood volume from the capacitance vessels to the central venous system, further augmenting right ventricular preload.8 The combined function of the thoracic pump and skeletal pump mechanism is essential for normal circulatory function.
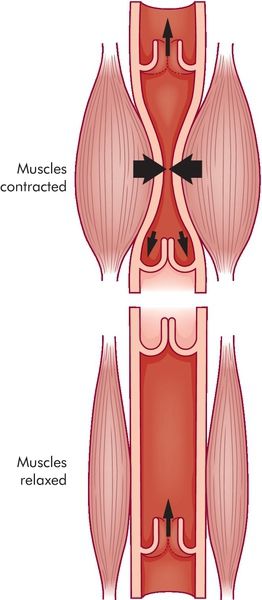
Venous Return
Key to appreciating the effects of ventilation and disease states on clinical hemodynamics is an understanding of venous return. The venous (capacitance) system comprises highly distensible vessels that hold approximately 70% of the body’s blood volume (Figure 14-3). This volume can be classified as either “unstressed” volume or “stressed” volume. Unstressed volume represents the largest volume of blood in the venous reservoir that is under minimal pressure.4 The stressed volume accounts for 30% of the total blood volume and is the venous volume under pressure resulting from increase in vascular tone or pressure surrounding the venous system.3,4,9–11 Unstressed volume can be converted to stressed volume as vascular tone or pressure surrounding the venous system increases. Vascular tone may be increased through the effects of endogenous catecholamines or the administration of exogenous (vasopressor) agents.2,3,12
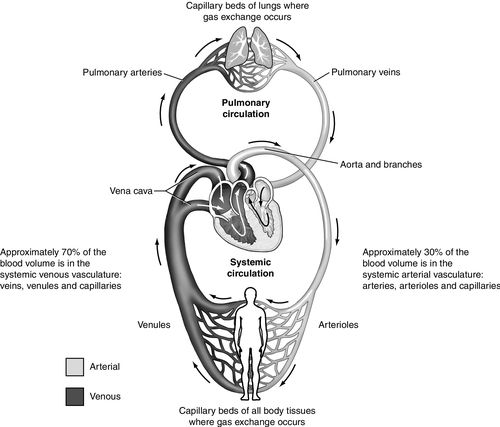
The mean systemic filling pressure (also called the mean circulatory filling pressure) is generated from the stressed volume and it largely determines the blood flow to the right side of the heart, specifically the right atrium. This concept was illustrated by Magder (Figure 14-4) as a reservoir with a drain in the side. The volume above the drain represented stressed volume, and the volume under the opening of the drain represented unstressed volume. In this model, the stressed volume (mean systemic filling pressure), combined with right atrial pressure and resistance to venous return, determines blood flow back to the heart.4
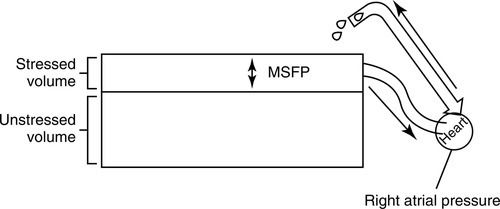
Right atrial pressure can impact venous return relative to the mean systemic filling pressure. This is the pressure of the venous blood volume that returns to the right heart. Normally, right atrial pressure is the lower value, allowing for forward flow from the venous circulation into the right atrium. The normal gradient from the venous system to the right atrium is approximately 4 to 8 millimeters of mercury (mm Hg). Venous return is maximal when right atrial pressure equals zero.2–4,7,13 Conditions that improve venous return include those that increase the stressed volume and decrease venous compliance, resistance, and right atrial pressure. Conditions that increase right heart afterload such as positive pressure ventilation, positive end-expiratory pressure (PEEP), or right ventricular heart failure will increase right atrial pressure relative to mean systemic filling pressure, thus reducing venous return.13
The skeletal pump described above may contribute to the conversion of unstressed volume to stressed volume. Patients who are immobile lose the skeletal pump contribution, resulting in venous or lymphatic stasis and edema. Early mobility in the critically ill ventilated patient may help limit the effects of immobility through restoration of the skeletal pump mechanism.8
Circulating Blood Volume
Conditions that alter vascular volume may also impact venous return. Hypovolemia, regardless of cause, reduces circulating vascular volume, venous return, and, eventually, cardiac output. Compensatory mechanisms for decreased preload, for example, tachycardia and vasoconstriction, have time-limited effectiveness.3,7,14 Conversely, hypervolemia may increase venous return. Hypervolemia may occur as a result of overzealous fluid resuscitation. Although usually well tolerated with normal cardiac function, it will be detrimental with left ventricular failure.3,7,14–18
Guyton described the interaction of venous return and cardiac function through the illustration of a venous return curve combined with the cardiac function curve, or Starling curve (Figure 14-5).9–11,16 The venous return curve represents venous flow (stressed volume) from the reservoir to the right heart (atrium). In this relationship, the Starling curve represents cardiac function and is plotted against right atrial pressure. The intersection of these curves provides the working or optimal value for this relationship (see Figure 14-5, point A).4,10,11,16 This relationship can change, depending on increases or decreases in venous return or subsequent shifts in cardiac function. Conditions such as hypovolemia or venodilation may reduce venous return (stressed volume) shifting the curve to the left (see Figure 14-5, from point A to point B or C),2–4,7,13 whereas increases in stressed volume (vasoconstriction) or fluid administration may increase venous return shifting the curve to the right (see Figure 14-5, point C or B back to A). These shifts can have subsequent effects on cardiac performance as well.2,3,7,9 Although combinations of these volume and pressure interactions are many, this chapter will focus on hemodynamic interactions associated with positive pressure ventilation and PEEP.
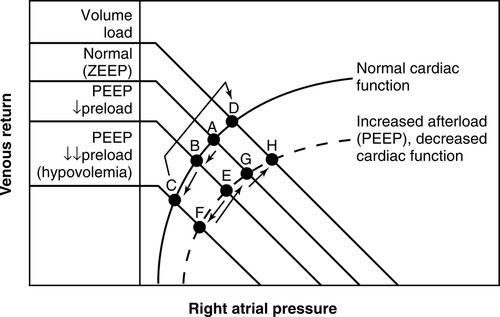
Positive Pressure Ventilation
The implementation of positive pressure mechanical ventilation alters the complementary physiologic balance between normal breathing and hemodynamic function (see Figure 14-1). During a positive pressure breath, gas flow enters the trachea, bronchi, bronchioles, and alveoli filling from the central airways to the peripheral alveoli.1,3,19 This sequence is opposite to that of physiologic breathing, as described above. Because of this, peripheral alveoli may be under-filled, depending on the tidal volume used in relation to the patient’s lung capacity.
The hemodynamic effects of positive pressure ventilation result from alteration in the normal relationship between physiologic ventilation and cardiovascular function. These changes may affect venous return as well as cardiac function.1–3,6,18,20 Additionally, they may be further determined by underlying cardiopulmonary pathophysiology. Each of these factors will be discussed further.
Venous Return and Positive Pressure Ventilation
Venous return is determined primarily by the gradient between mean systemic filling pressure and the right atrial pressure.4,10 Both positive pressure ventilation and PEEP have the potential to reduce venous return by increasing pressure on the superior and inferior vena cava in the thoracic cavity thereby reducing flow to the right atrium (see Figure 14-1).2,13,21–24 Increases in venous return through an increase in mean systemic filling pressure may occur as a result of neurohumoral responsiveness, vasopressor administration, or volume administration.1–3,7,19,25
The degree to which venous return is impacted by positive pressure ventilation is dependent on various factors. Such factors may include intravascular volume status, right heart function, and positive pressure increases in intrathoracic pressure transmitted to the heart and vascular structures. Intrathoracic pressure influence is complex because it is determined by chest wall and lung mechanics, as well as the ventilation mode parameters. Pulmonary mechanics include airway resistance, compliance, and elastance.18,26,27 Ventilation parameters include tidal volume, PEEP level, and use of inverse ratio ventilation, or spontaneous breathing modes such as pressure-support and biphasic ventilation. Each of these factors, individually or in combination, will affect patients differently. When possible, this impact should be anticipated to prevent hemodynamic compromise. Each of these factors will be discussed further.
Positive Pressure Ventilation and Effect on the Right Heart
Right Heart Preload
Alterations in right heart hemodynamics associated with positive pressure ventilation primarily include reduction in preload and increase in afterload. Preload is impacted through reduction of venous return by mechanisms already discussed. Although reduction in preload as a result of decreased venous return is often cited as the cause of diminished right heart output, other evidence suggests that increased afterload on the right ventricle plays a more significant role.2,12,13,28–30
Ventilation and Perfusion Ratio
From a physiologic standpoint, total lung ventilation (V) and perfusion (Q) are equally matched with the V/Q ratio equal to 0.8 to 1. In reality, the V/Q ratio varies, depending on the lung region, patient position, mechanical ventilation, and pathophysiologic alterations in ventilation, perfusion, or both.
Lung Zone Pressures
In 1964, West described the relationship between alveolar, arterial, and venous pressures in the lung, progressing from the apex to base when a person is in an upright position (Figure 14-6, A).31 In this model, Zone 1 is the upper third of the lung, where ventilation is greater than perfusion. Zone 2 is the middle portion of the lung, where ventilation and perfusion are more equally matched. Zone 3 is the bottom portion of the lung, where perfusion is greater than ventilation.31 Although often described as progressing from the apices to the base of the lung, these zones progress from the anterior to the posterior lung when the patient is lying in the supine position.
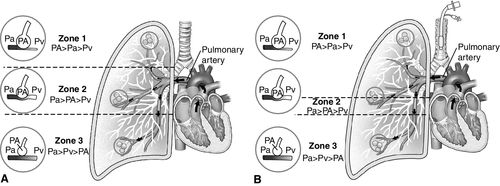
Even though physiologic variations in the V/Q ratio may occur as described above, pathophysiologic changes in the V/Q ratio also occur. Ventilation in excess of perfusion, or perfusion in excess of ventilation, results in a V/Q mismatch. When these imbalances occur to the extreme, they result in dead space or shunt conditions. Dead space ventilation conditions occur when the alveoli are ventilated, but there is no perfusion through the associated alveolar capillaries. Pulmonary embolism is a classic example of dead space ventilation (see Chapter 8, Figure 8-7). A shunt condition results when alveoli are not ventilated (fluid filled or collapsed), but perfusion to the alveoli is relatively normal. Pulmonary edema, pneumonia, or ARDS may result in shunt conditions. Clinically, both dead space and shunt conditions may co-exist simultaneously. Patients with ARDS have alveolar collapse (shunt) as well as perfusion abnormalities that result in dead space conditions. This is further complicated by the application of positive pressure ventilation.
The normal ventilation perfusion relationships described above can be altered by positive pressure ventilation and PEEP as alveolar overdistention may increase Zone 1 conditions into Zone 2 as shown in Figure 14-6, B. In addition to causing alveolar damage, overdistention may compress adjacent pulmonary capillaries, limiting blood flow to overdistended alveoli and resulting in V/Q mismatch or dead space conditions. This compression also redirects capillary blood flow in the direction of least resistance toward underventilated or collapsed alveoli. Perfusion will increase to these derecruited alveolar areas without the benefit of matched ventilation, resulting in worsening shunt (Figure 14-7).13,24,32–35
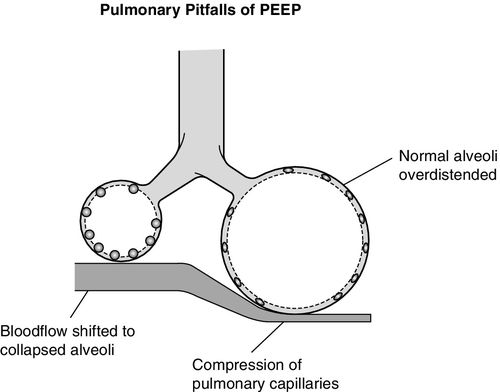
Right Ventricular Afterload
In addition to mechanical vasoconstriction associated with alveolar overdistention, hypoxic vasoconstriction in the lung may further increase pulmonary vascular resistance and right ventricular afterload. Hypoxic vasoconstriction, mediator-induced vasoconstriction, and microvascular clots in the pulmonary circulation contribute to the right ventricular (RV) dysfunction often seen in ARDS.36–38 These concepts will be discussed later in this chapter.
RV dilation may also negatively impact coronary artery blood flow, as high wall tension compresses the right coronary artery reducing blood flow to the myocardium. Increased RV afterload associated with vasoconstriction and positive pressure ventilation may further increase myocardial oxygen demand, leading to ischemia and infarction.2,4,28,29,39–42
Therapeutic strategies to reduce right heart afterload include the use of recruitment strategies to open collapsed alveoli to improve ventilation or oxygenation and reverse hypoxic vasoconstriction. Also, pulmonary vasodilators such as inhaled prostacyclin and nitric oxide may be used to reduce pulmonary vascular resistance and decrease afterload.13,30,43–45 These inhaled agents are distributed to the best-ventilated regions of the lung. They cross the alveolar capillary membrane into the adjacent capillaries, causing vasodilation and improved perfusion to the ventilated alveoli. This, in turn, improves the V/Q matching of these units, allowing for better gas exchange.46,47 Although these changes may be beneficial from a physiologic standpoint, reductions in mortality have not been demonstrated, even with severe hypoxemia.48
Positive Pressure Ventilation and Effect on the Left Heart
Left Heart Preload
The impact of positive pressure ventilation and PEEP on the left heart is caused by the downstream effects of intrathoracic pressure on the right heart. Left ventricular (LV) preload is reduced as a result of reduced RV output. Though positive pressure ventilation may expel some blood from the pulmonary circulation into the left atrium contributing to LV preload, this does not fully compensate for the reductions in left heart preload associated with the reduction in RV output to the left side of the heart.1,2,4,15,17,18,29,39,49
Similarly, ventricular interdependence (Figure 14-8, A) contributes to reduction of LV preload. As RV afterload increases, the right ventricle becomes distended, displacing the shared ventricular septum into the left ventricle, reducing the filling capacity of the left ventricle (see Figure 14-8, B). This decreases the diastolic filling capacity, subsequently reducing LV output.2,3,13,39,50
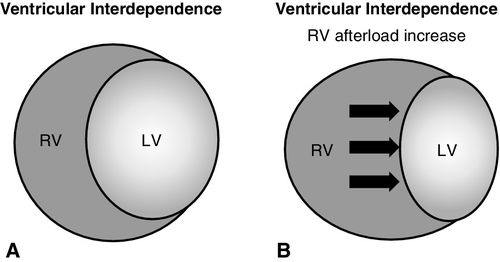
Left Ventricular Afterload
During spontaneous ventilation, intrathoracic pressure decreases more so than the intraluminal pressure of the aortic root, since a portion of the aorta is located outside of the thoracic cavity. LV afterload is greater during spontaneous inspiration than during spontaneous expiration. Additionally, RV filling during spontaneous inspiration results in transient displacement of the ventricular septum into the left ventricle, reducing LV size, compliance, and preload.2,4,13 This does not negatively impact cardiac output in those with normal LV function, but in patients with LV failure, increased work of spontaneous breathing may further increase myocardial oxygen demand, which results in ischemia.1,19,51 This factor should be considered during ventilator liberation as the patient transitions from positive pressure ventilator support to spontaneous breathing. In patients with impaired ventricular function, transition from full support to spontaneous breathing may increase LV workload. Spontaneous breathing augments venous return to the right heart (RV preload), which results in an increase in left heart preload as well as in LV afterload. Increased work of breathing may also increase myocardial oxygen demand.1,3,17–19
During positive pressure ventilation, the transient increase in pressure during the inspiratory phase results in higher intrathoracic pressure relative to the extrathoracic pressure. Higher intrathoracic pressure applied to the mediastinum and intrathoracic aorta results in an elevated extramural pressure relative to the intraluminal pressure of the aorta and the circulatory system outside of the thorax at atmospheric pressure. This pressure gradient transiently reduces LV afterload and improves LV output.2,4,13,52–54 With the application of PEEP, intrathoracic pressure remains elevated during the expiratory phase as well, sustaining the benefit of some afterload reduction during the exhalation phase of the positive pressure ventilation breath. Although this application of PEEP should theoretically improve cardiac output in the normal heart, it does not result in appreciable improvements. This may be due to co-existing reductions in RV preload, increased RV afterload and subsequent decrease in LV preload.3 In LV failure, however, there is an increased sensitivity to preload reduction. PEEP may, therefore, have a beneficial effect in improving LV function by reducing LV preload and LV afterload in a similar fashion to vasodilators in heart failure.1,2,4,13,29
Cardiac Contractility
The effects of positive pressure ventilation and PEEP on preload and afterload are well described. However, the impact on cardiac contractility, the third component of stroke volume is less clear. Inotropic function may be indirectly affected by reduction in coronary artery blood flow as well as release of humoral mediators as a result of positive pressure ventilation and PEEP. Increase in RV afterload may reduce RV ejection fraction and worsen cor pulmonale associated with ARDS.13,29,30,42,55 LV contractility may be reduced as overdistention of the right ventricle impacts the left ventricle through the septum and ventricular interdependence. Conversely, PEEP may improve contractility in the failing ventricle by reducing preload and afterload as described above.13
Heart Rate
Reductions in stroke volume are normally compensated by an increase in heart rate to maintain physiologic cardiac output. This is primarily mediated through the carotid baroreceptors (see Chapter 1, Figure 1-16). With positive pressure ventilation and PEEP, this chronotropic compensatory response appears to be blunted.13 Despite reduction in stroke volume, some patients maintain normal or even reduced heart rates. Activation of pulmonary stretch receptors may be implicated in this response. The classic Hering-Breur reflex results in a transient increase in heart rate during inspiration. This is often the underlying cause of sinus arrhythmia. Inflation with higher pressures, however, may result in lower heart rate or bradycardia. Such may be the case with positive pressure ventilation and PEEP. This is clinically important, since the expected tachycardic response traditionally associated with reduced stroke volume may be blunted and not be a reliable hemodynamic finding in these patients.
Positive End-Expiratory Pressure
PEEP is a positive pressure applied through the ventilator, maintained during the expiratory phase of the ventilator breath to prevent alveolar collapse. Under normal physiologic conditions, alveolar collapse is prevented during exhalation by the volume of air remaining within the lungs; this is known as the functional residual capacity or FRC. Surfactant secreted by the type II alveolar cells reduces alveolar surface tension, resisting alveolar collapse associated with normal elasticity. Certain disease conditions such as ARDS may disrupt this normal function resulting in alveolar collapse.36,37,56
Atelectasis may result in alveolar collapse from hypoventilation. Resolution may be achieved in spontaneously breathing patients with deep breathing exercises or incentive spirometry. During mechanical ventilation, adding PEEP may resolve or prevent atelectasis. The level of PEEP required to achieve this goal is patient-specific; however, PEEP levels up to 5 centimeters of water (cm H2O) are often employed empirically in the intubated patient to prevent atelectasis.57 In more severe conditions such as ARDS, the alveoli fill with proteinaceous fluid resulting from immune-mediated injury to the alveolar capillary membrane.36,56,58,59 This alteration in permeability leads to pulmonary edema that reduces alveolar gas exchange and inactivates surfactant, resulting in both fluid filled and collapsed alveoli.36,56,59 Edema is most prominent in dependent regions of the lung, with the edema pattern shifting, depending on patient position.56,58 Further, the weight of the fluid-filled lung tissue may compress the regions below them, thus worsening the alveolar collapse. This collapse may range in severity and result in varying degrees of shunt.
In these conditions, PEEP may be used to prevent end-expiratory alveolar collapse after delivery of the ventilator breath or, at higher levels, used to assist in the lung recruitment. Many patients are placed on low levels of physiologic PEEP (5 cm H2O) as a standard setting on mechanical ventilation. This level does not impact hemodynamics but may prevent or improve atelectasis.57 At higher levels, PEEP may affect hemodynamics in a similar fashion to positive pressure ventilation as discussed above. The terms “low” PEEP and “high” PEEP are not defined consistently in the literature. A recent Cochrane review compared high versus low PEEP in mechanically ventilated adult patients with acute lung injury and ARDS and included seven studies in the meta-analysis. Across all studies, the definition of low versus high PEEP differed.60 In most cases, low PEEP was less that 10 cm H2O, and high PEEP was greater than 10 cm H2O. The absolute PEEP value is less likely to predict the hemodynamic effect. Rather, it is the combination of PEEP, lung mechanics, and vascular volume status that determine how a patient will respond to the set PEEP. It is important to remember that unlike the episodic pressure changes associated with the inspiratory and expiratory phases of the positive pressure ventilation breath, PEEP is maintained during exhalation as well.23,32,61,62 Therefore, hemodynamic changes will be sustained or altered, depending on the level of PEEP and for any increases or decreases in the PEEP settings.
Positive End-Expiratory Pressure and Venous Return
When PEEP is not applied, a condition of zero PEEP (ZEEP) is assumed. The relationship of venous return to cardiac output (see Figure 14-5, point A) is maintained.
PEEP and Low Preload: With the addition of PEEP, the venous return curve may be shifted to the left (see Figure 14-5, point B) reducing preload. In the face of pre-existing hypovolemia, the addition of PEEP may markedly reduce preload (see Figure 14-5, point C). Volume administration can restore the normal relationship (back to point A) or to above normal levels (see Figure 14-5, point D).
PEEP and Increased Afterload: However, PEEP may also shift the cardiac output curve to the right as a result of increased afterload.3,4,13,33 PEEP has combination hemodynamic effects of both RV preload reduction and increased RV afterload, thus PEEP can reduce overall cardiac output (see Figure 14-5, point E).
PEEP and Mean Systemic Filling Pressure: In the face of hypovolemia, venous return may decrease and subsequent cardiac function may become markedly depressed (see Figure 14-5, point F). However, PEEP may also increase the mean systemic filling pressure of the venous return by increasing stressed volume through activation of the sympathoadrenal response.2,3,7,13 This would increase venous return and maintain the venous return–cardiac function relationship to near normal (see Figure 14-5, point G). Administration of fluid volume may further augment venous return (see Figure 14-5, point H). The concepts of mean systemic filling pressure and stressed volume are illustrated in Figures 14-3 and 14-4.
Reductions in venous return related to PEEP seems to be effected by vascular volume status. For patients who are hypovolemic, increases in PEEP may result in compression of the superior vena cava as demonstrated by echocardiography.28,40,63 Patients who are euvolemic or hypervolemic may not experience reduction in venous return, even at PEEP level of up to 20 cm H20.2,3,13,33,42,64,65 Conversely, those who have underlying hypovolemia may experience hypotension resulting from reduced cardiac preload and increased afterload, as previously discussed.
Positive End-Expiratory Pressure and Right Heart Function
Reductions in RV preload from PEEP are the result of reduced venous return. However, PEEP may also impact RV afterload by decreasing or increasing pulmonary vascular resistance. In the collapsed regions of the lung, pulmonary vascular resistance is increased because of hypoxic vasoconstriction as well as compression of alveolar capillaries by fluid-filled alveoli.2,4,13,30,54,66 When positive pressure is applied to re-expand the collapsed regions, it improves gas exchange and oxygenation, thus reversing hypoxic vasoconstriction and reducing pulmonary vascular resistance. Additionally, PEEP reduces pulmonary vascular resistance by inflating the collapsed regions, restoring the functional residual capacity (FRC) and opening small pulmonary capillaries.13,38,43,67,68 At lung volumes below the FRC, vascular resistance is high. As alveoli are re-expanded to normal FRC from residual volume, the expansion of the alveoli opens capillaries, improving alveolar capillary blood flow and reducing pulmonary vascular resistance. These two mechanisms highlight the beneficial impact of PEEP in improving RV function through afterload reduction.2,3 It is important to note however that overinflation of alveoli toward total lung capacity will cause capillary compression increasing pulmonary vascular resistance and RV afterload. This is an important consideration, as increased RV afterload combined with decreased preload will reduce overall cardiac output.2–4,29,33
Although some studies have shown that RV function is preserved with PEEP levels up to 20 cm H2O or greater, others have noted reduction of RV function and the development of cor pulmonale with the use of PEEP. This may be even more prominent in patients with ARDS, when ARDS is associated with RV afterload elevation from alveolar collapse, pulmonary vasoconstriction, and pulmonary capillary microthrombosis. Lung-protective strategies aimed at reducing overdistention (low stretch) and recruitment are desirable to reduce RV afterload and improve cardiac output.30,33,42,55,69–71
Positive End-Expiratory Pressure and Left Heart Function
Left heart preload may be reduced by PEEP because of reduction in RV output from the mechanism described above. Additionally, ventricular interdependence associated with a shared ventricular septum results in decreased LV preload resulting from displacement of the septum into the left ventricle from RV dilation (Figure 14-8, B). For patients with failing LV function, PEEP, similar to venodilators, may improve function by reducing LV preload and by reducing afterload through an increase in juxtacardiac pressure in relation to extrathoracic pressure. This gradient improves the ejection of blood from the left ventricle.4,7,13,29,53,72
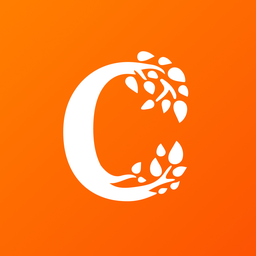
Full access? Get Clinical Tree
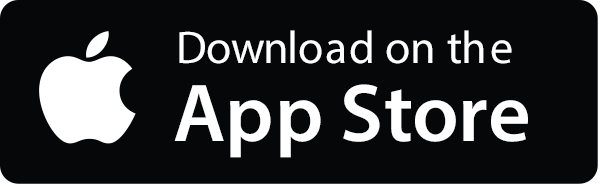
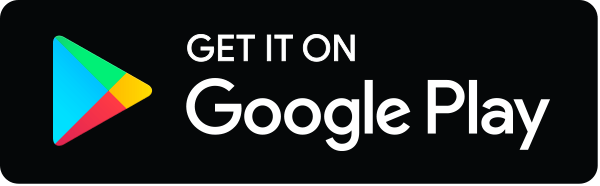