Karl D. Hillenbrand,, Robert H. Thiele Thoracic surgery is a rapidly changing surgical subspecialty that presents the anesthesiology community with a myriad of challenges, one of which is hemodynamic monitoring. Thoracic surgical patients range from relatively healthy adults with refractory gastroesophageal reflux in need of a laparoscopic Nissen fundoplication, to patients with severe atherosclerosis and chronic obstructive pulmonary disease needing a pneumonectomy for cancer resection, to patients in need of lung transplantation. The 30-day mortality for this population ranges from less than 1% for sublobar resections, to almost 4% for pneumonectomies, and to 6% for lung transplantation. Complicating hemodynamic monitoring and management of this population is the deep interdependence between the cardiac and pulmonary systems. The combination of comorbidities, invasiveness of the procedure, impact of thoracic surgery on hemodynamics, and challenges associated with monitoring make thoracic surgical procedures some of the most difficult and rewarding procedures that an anesthesiologist will manage. The purpose of this chapter is to review the hemodynamic monitoring devices available to assist in the management of this diverse population, with a particular focus on blood pressure monitoring, cardiac output, and fluid responsiveness. Also included are central venous pressure, pulmonary artery pressure, and extravascular lung water. blood pressure monitoring; central venous pressure; pulmonary artery pressure; cardiac output monitoring; fluid responsiveness; extravascular lung water Thoracic surgery is a rapidly changing surgical subspecialty that presents the anesthesiology community with a myriad of challenges, one of which is hemodynamic monitoring. Thoracic surgical patients range from relatively healthy adults with refractory gastroesophageal reflux in need of a laparoscopic Nissen fundoplication, to patients with severe atherosclerosis and chronic obstructive pulmonary disease needing a pneumonectomy for cancer resection, to patients in need of lung transplantation. The 30-day mortality for this population ranges from less than 1% for wedge resections, to almost 4% for pneumonectomies, and to 6% for lung transplantation.1,2 By comparison, 30-day mortality for coronary artery bypass grafting is approximately 1%.3 Complicating hemodynamic monitoring and management of this population is the deep interdependence between the cardiac and pulmonary systems. The combination of comorbidities, invasiveness of the procedure, impact of thoracic surgery on oxygenation and hemodynamics, and challenges associated with monitoring make thoracic surgical procedures some of the most challenging and rewarding procedures that an anesthesiologist will manage. Oscillometric blood pressure measurement is by far the most popular method of measuring blood pressure in the operating room (OR) in developed countries—at academic medical centers in the United States, 84% of surgical patients receive oscillometry alone.4 The engineering principles behind oscillometry were first described in 1876 by Marey.5 With the oscillometric technique, a cuff is inflated to a pressure higher than the systolic blood pressure (SBP), which occludes all flow to the extremity. At this occlusion pressure, no (Korotkoff) sounds are produced on auscultation, and pulsatile pressure transmitted to the cuff is minimal (Fig. 12.1). Gradually (or stepwise), the cuff is deflated until the pressure is below the diastolic blood pressure (DBP). As the pressure within the cuff is deflated, blood begins to flow into the extremity, which produces oscillations in the cuff pressure, which can be measured and recorded. As noted by Posey et al., the pressure within the cuff at which oscillations are maximal is closely correlated with mean arterial pressure (MAP).6 The algorithms used to determine SBP and DBP are propriety to each manufacturer. The introduction of oscillometric blood pressure measurement offered to the anesthesiologists many advantages in an OR environment when compared with the classic auscultatory noninvasive method: it can be automated (freeing up the anesthesia provider to perform other tasks), it requires significantly less skill and training to use, it can be more forgiving in patient positions in which frequent access to a distal artery for auscultation would prove difficult or impossible, and it is easier to use in a noisy OR environment. Unlike intra arterial catheters, which are site independent (measured blood pressure does not change with movement of the extremity as long as the level of the transducer is not changed), oscillometric cuffs measure blood pressure at a specific location, irrespective of its location relative to the right atrium. The anesthesiologist caring for the thoracic surgical patient must therefore take care to account for cuff position relative to the organ of interest when interpreting blood pressure values. Given the frequent use of lateral positioning during thoracic procedures, the extremity on which the blood pressure cuff is placed may be higher or lower than the heart and brain, which are the primary organs of interest for blood pressure management. The arterial system is valve-less, and the pressure within it will therefore fall linearly with increasing height against gravity. However, given that blood pressure is measured in mm Hg and mercury is far denser than blood, a correction factor (0.74 mm Hg lower for every 1 cm) must be applied to measure the impact of these changes. Moving the blood pressure cuff from the dependent to nondependent arm in a patient in the lateral position can significantly change the reported blood pressure because of hydrostatic effects,7 despite the fact that actual blood pressure in the areas of interest (brain, heart) has not changed at all (Fig. 12.2). Oscillometry has been extensively compared with the gold standard of intraarterial catheterization. A single center, retrospective analysis of 15,310 noncardiac anesthetics in which the patient had both intraarterial catheters placed as well as oscillometry used found that the standard deviation of MAP was ± 12.5 mm Hg around a MAP of 75 mm Hg, when oscillometry was most accurate—this equates to 95% confidence intervals of approximately ± 25 mm Hg (Bland-Altman analysis). When MAP deviated substantially, the performance of the oscillometric technique decreased even further.8 In 1963, Pressman and Newgard published the first description of what would become artery applanation tonometry.9 This was the first development of a continuous form of noninvasive blood pressure monitoring, and the concept was advanced by O’Rourke.10 Applanation tonometry involves the flattening (applanation) of a superficial artery, most commonly the radial, with a pressure sensing device.11 Only arteries that can be compressed (e.g., a radial artery) can be assessed. When the artery is compressed enough to reach a flattened state, the pressure sensed by the detector is similar to the intraarterial pressure, and the resulting pressure waveform closely mimics the true intraarterial pressure waveform.12 Tonometers are incapable of making absolute pressure measurements and thus need to be calibrated against another device (e.g., blood pressure cuff).12 The use of frequency domain mathematical analysis (e.g., fast Fourier transformation) allows a peripheral artery tracing to be “transformed” into an estimate of central arterial blood pressure using what is referred to as a transfer function.13 If measurements are taken at a “large” artery, applanation tonometry is relatively resistant to confounding variables that can disrupt other noninvasive continuous blood pressure measurement methodologies (see later) such as vasoconstriction. Applanation tonometry has been validated in over 1600 patients, including over 15,000 individual blood pressure measurements14 but is clinically impractical in the OR because it requires unrestricted access to the wrist for placement of the pen-like “tonometer.” Because the underlying technology is sound, but the practical implementation is cumbersome, efforts have been made to miniaturize applanation tonometers for utility in the OR environment. Testing by multiple groups has revealed relatively low bias (6.3 mm Hg, range 4.8–12.6 mm Hg)15–19 and limits of agreement (approximately ± 12.4 mm Hg, range ± 9.4 to ± 24.7 mm Hg) that are at least comparable, if not slightly better than noninvasive devices generally.20 A recent large metaanalysis has cast some doubt onto the accuracy of tonometers in patients who have cardiovascular disease. “The results from this metaanalysis found that inaccuracy and imprecision of continuous noninvasive arterial pressure monitoring devices are larger than what was defined as acceptable. This may have implications for clinical situations where continuous noninvasive arterial pressure is being used for patient care decisions.”21 First described by Penaz in 1973, volume clamping is a method of noninvasive blood pressure measurement that can provide beat-to-beat information in real time.22 It involves placement of an ultrafast, inflatable cuff upstream of a finger-based infrared sensor and emitter. By using a feedback loop between the cuff and the infrared sensor, the cuff is inflated and deflated cyclically such that the intensity of the infrared radiation is nearly constant (i.e., the blood volume of the finger is unchanged). In theory, if the pressure in the cuff exactly matches the arterial blood pressure, changes in finger blood volume (and absorbance of infrared light) are minimal. Thus the pressure waveform required to maintain minimal variation in infrared absorbance is thought to closely approximate arterial blood pressure (Fig. 12.3). These devices need to be periodically calibrated by taking advantage of the fact that detected pulsatility distal to a cuff is maximal near MAP (discussed earlier during oscillometry). When external cuff pressure is close to blood pressure, the arterial wall experiences minimal tension and is said to exist in an “unloaded” state. Commercially available devices that use the volume-clamp technique differ in the algorithms used to achieve vascular unloading. Given the dependence of the volume clamp technique on small vessel, distal extremity perfusion, it is susceptible to periods of hypoperfusion6,7 and poor blood flow as can be seen in shock, and hypothermia23 will impact the accuracy of the blood pressure measurements. Individual studies looking at agreement between volume clamping and applanation tonometry in a variety of settings have been mixed; and in 2014, a metaanalysis of both of these techniques demonstrated an accuracy that was slightly worse than noninvasive technique overall.20 Of course, this decrease in accuracy needs to be counterbalanced by the continuous measurements provided by these devices, which is an important advantage. As the technology improves, its potential to provide beat-to-beat blood pressure values noninvasively is tantalizing and has significant implications in large surgeries with significant fluid shifts and hemodynamic swings, such as thoracic surgery. When continuous, accurate blood pressure monitoring is indicated, intraarterial catheterization is most widely used. This technique is particularly useful in thoracic surgery patients because it also permits sampling for arterial blood gas analysis. A detailed review of arterial physiology is beyond the scope of this chapter and is available elsewhere.24 Three key points related to intraarterial blood pressure monitoring deserve mentioning. First, a basic understanding of the engineering principles behind intraarterial blood pressure monitoring is necessary to use the technology properly. Intraarterial blood pressure monitors use a “Wheatstone bridge” strain gauge to estimate the intraarterial pressure. This pressure is transmitted through an uninterrupted column of fluid that begins with an intraarterial catheter and extends to the strain gauge, where the column terminates.25,26 The physical characteristics (length, diameter, distensibility) of the tubing used to connect the catheter to the strain gauge impact the appearance of the pressure waveform. To accurately measure relevant features of the waveform (systolic and diastolic pressure), it is essential that the measurement system has an appropriate dynamic response—this can be determined by measuring the natural frequency (fn, the oscillating frequency after application of a pressure pulse) and the damping coefficient (ζ, the time it takes an oscillating waveform to decay). Both fn and ζ can be measured using the “flush test”—fn is the inverse of the time between two peaks (in s-1), and ζ is the ratio of two successive amplitudes (A2/A1). By plotting fn and ζ on a “dynamic response map,” the adequacy of the measurement system can be ensured27,28 (Fig. 12.4). In general, clinicians should use rigid, short tubing systems to connect the pressure monitor to the intraarterial catheter, and take great care to avoid air bubbles. The compressible air bubbles results in damping of the arterial tracing. Of note, the MAP reading will not be affected by inadequate dynamic response, only the shape of the waveform and the resultant estimates of systolic and diastolic pressure. Second, the shape of the blood pressure waveform is highly dependent on the physical characteristics of the cardiovascular system, as well as the measurement location (Fig. 12.5). Rigid, noncompliant vessels will alter the arterial waveform, which is a combination of forward and backwards (from reflection sites) traveling wavefronts.24 A common clinical mistake is to see an extremely high systolic pressure and attribute it to “whip” (i.e., an underdamped system), when in reality the dynamic response of the arterial measurement system is fine. This is common in patients with significant cardiovascular disease and atherosclerosis, especially when pressure is measured at a peripheral site (e.g., radial artery). Third, although the infection rate of intraarterial catheters is low (approximately 1.7 per 1000 catheter days), it is comparable to the infection rate of nontunneled, centrally inserted central venous catheters (2.7 per 1000 catheter days).29–31 The risk of infection can be lowered through sterile insertion technique and the use of a chlorhexidine-impregnated dressing.32 Traditionally, central venous pressure (CVP) was thought to represent “preload” and has served as a guide for volume administration in various goal-directed therapy algorithms.33 Rigorous physiologic research has subsequently demonstrated that there is no relationship between CVP and blood volume, no relationship between changes in CVP and changes in blood volume, and that CVP has no relationship with fluid responsiveness.34 In addition to the complex chain of physiologic interactions linking the systemic venous system to left ventricular end diastolic volume (including left ventricular compliance, intrathoracic pressure, pulmonic and mitral valve function, pulmonary vascular resistance, and right ventricular function),35,36 healthcare practitioners themselves show wide variation in transducer placement,37 adding a component of technical error to an already complex measurement. Complicating matters in the thoracic surgery population is the impact of lateral positioning on CVP, which should be measured at the level of the right atrium—both clinical and imaging studies have confirmed that great attention must be paid to transducer height when moving to the lateral position.38–40 Given the growing emphasis on dynamic indicators of volume status (see “Fluid Responsiveness” later), and the known risks of central venous catheter placement, CVP is rarely used as a physiologic guide at the authors’ institution. Unfortunately, large datasets on usage rates are not currently available.41 One relatively recent use of CVP monitoring that may be useful in particularly high risk thoracic surgical patients (e.g., lung transplantation) is the pulmonary artery pulsatility index (PAPi) (Fig. 12.6). The idea behind PAPi is that combining data from the systemic venous system and pulmonary arteries may give practitioners some insight into right ventricular function.42 As described earlier, unlike the CVP alone, which is distantly related to left ventricular filling, the systemic venous system and pulmonary artery are separated by fewer anatomic structures. PAPi is defined as pulmonary artery systolic pressure—pulmonary artery diastolic pressure/CVP and essentially indexes the pulmonary artery pulse pressure (which is related to right ventricular stroke work) to CVP. A normally functioning right ventricle can produce adequate cardiac output without the need for high right atrial pressures. In contrast, a failing right ventricle requires higher and higher right atrial pressure to generate adequate cardiac output. Although use of this metric is still relatively new, it appears to predict right ventricular dysfunction in a variety of patient populations. These include after insertion of left ventricular assist device,43 emergency transcatheter aortic valve replacement,44 as well as predicting adverse events in advanced heart failure patients45 and with right heart failure in particular,46 and may be useful in select thoracic surgery patient populations. Pulmonary artery catheters (PACs), in addition to providing cardiac output (CO) information, can provide contentious real-time information on CVP and pulmonary arterial pressures. This can be especially important in the thoracic surgical patient who may present with, develop, or worsen intraoperatively pulmonary hypertension because of the pathology of their lung disease or during the intraoperative course. The data provided by a PAC can be interpreted to give some information regarding the function of the patient’s right ventricle. A PAC may be especially valuable in the patient with pulmonary hypertension, as Doppler ultrasound can give inaccurate values for pulmonary artery pressure.47,48 Any potential benefits to PAC placement must be balanced against its risks, including pulmonary artery rupture (especially in patients with pulmonary arterial hypertension), damage to cardiac valves, endocarditis, arrythmia, including ventricular fibrillation, catheter knotting, intravascular thrombosis, vascular stenosis, and catheter-related bloodstream infection. In addition, multiple studies49–51 have failed to demonstrate a mortality benefit to PAC use in critically ill intensive care unit (ICU) patients, however, these studies did not focus on thoracic surgical patients specifically. Because of the lack of proven benefit and the continued improvement in noninvasive monitoring techniques, a sharp drop-off in the use of PACs has been seen in North American ICUs,52,53 although this trend may be reversing.54 More recent metaanalyses have demonstrated a mortality benefit with the use of PACs in critically ill patients undergoing major surgery.55,56 Although the fate of the PAC has yet to be determined, it remains a valuable tool available to the anesthesiologist especially when supporting patients with pulmonary hypertension or right heart dysfunction. In thoracic surgical populations, common usage of the PAC is typically limited to lung transplantation. The measurement of blood pressure, discussed earlier, is to a large extent conducted in an effort to gauge delivery of oxygen (DO2) to important organs, with blood pressure acting as a surrogate marker of end-organ perfusion. Blood pressure has the benefit of being easily and directly measurable, but it is an imperfect surrogate for oxygen delivery, which is of primary importance to the anesthesiologist. Although MAP is a critical component of global CO (and thus oxygen delivery globally) in the surgical setting, there is very little relationship between MAP and DO2, likely because of differences in systemic vascular resistance57 (Fig. 12.7). Thus an accurate measure of CO, which directly affects oxygen delivery, has long been a desired but elusive physiologic parameter for the anesthesiologist. The de facto gold standard for CO monitoring is currently the pulmonary artery thermodilution method, which was first published by Fegler in 1954.58 This technique is based on previous work using dilutional methods for monitoring CO involving dyes or other chemicals, such as lithium. An advantage of the thermodilution technique is its ability to be used repeatedly without concerns for increasing levels of a foreign substance within the body. Pulmonary artery thermodilution requires the placement of a PAC, as an accurate measurement requires the ability to measure temperature before and after the right ventricular outflow tract. After pulmonary artery placement is complete and verified, a known volume of an injectate (typically crystalloid solution) at a known temperature is injected briskly and mixes with the patient’s blood. A thermistor at the tip of PAC then measures the change in temperature over time, and the area under the curve is used to calculate the CO: the total thermal mass of the fluid bolus is known (based on temperature, volume, and specific heat of crystalloid solution), and a complex series of equations (known as the Stewart-Hamilton equations59) can be used to convert the temperature profile in the pulmonary artery to right ventricular output. From a qualitative sense, a high CO will produce a sharp but brief change in temperature as cold crystalloid races past the tip of the PAC, whereas in the setting of low CO, the temperature change will be more gradual and prolonged because the blood is moving more slowly and the cold fluid has more time to mix. Pulmonary artery thermodilution has limitations. It assumes all blood flow is forward and in series, at least on the right side of the heart. Both tricuspid regurgitation and intracardiac shunts can give erroneous CO values by having some of the thermal mass of the injectate be delayed in its presentation to the thermistor or bypass it all together. Flow must not be so slow nor so fast such that adequate mixing of the injectate and patient’s blood volume does not occur before the thermistor, and the temperature difference between the patient’s blood and injectate must be sufficient enough to induce a measurable change in temperature at the thermistor. Although the temperature of the injectate does not seem to change CO values measured, using a colder injectate (i.e., 0oC) does reduce the variability of the values obtained compared with using room temperature injectate at the cost of additional equipment. Similarly, injecting a larger volume of injectate can improve the reliability of the results.60 For more continuous feedback, the practitioner can decide to place a continuous cardiac output (CCO) PAC. With this method, the PAC contains a filament midway along the catheter that is periodically heated, warming the blood. This change in temperature is then measured by the thermistor at the tip of the PAC, and CO is calculated in a similar manner to the intermittent thermodilution method described earlier. Because the “thermal mass” associated with these temperature changes is smaller than the thermal mass of a 10 mL bolus of room temperature (or cold) crystalloid, a substantial number of readings must be taken and averaged over time—thus CCO PACs typically have a time delay of as many as 5 minutes which limits its clinical use in an acute setting.61–63 Whether or not CO measurement improves clinical outcomes is a matter of fierce debate, initiated by a series of large, randomized controlled trials (RCTs) that failed to show improvements in mortality when PACs were used in critically ill patients.51,64,65 Although questions about study design and protocol adherence plague these studies, the impact on PAC use is not debatable. By 2004, PAC insertions had fallen to 1.99 per 1000 medical admissions in the United States,52 with even more recent Canadian data suggesting a similar decrease in PAC use.53 There is some evidence that the trend toward decreasing PAC use is starting to reverse.54 Given the lack of high-quality data suggesting that PACs improve outcomes, and the increased appreciation for the risks and costs associated with their placement, substantial effort has been invested in the development of techniques to accurately measure CO without the need for the invasive use of a PAC. An obvious starting place would be to attempt to measure temperature changes somewhere other than the pulmonary artery, and transpulmonary thermodilution CO (TDCO) devices do just that. Room temperature crystalloid is injected into the right atrium, and temperature changes are measured in a large artery after the fluid bolus has travelled through the lungs and left atrium/ventricle. Transmission across these additional structures, as well as the fact that not all CO passes through the measurement site (e.g., femoral, axillary, or brachial artery) complicate the measurement. However, overall comparisons to both experimental gold standards, as well as thermodilution in animals and humans66–79 suggest that transpulmonary thermodilution measurements are likely to be noninferior to that of pulmonary artery thermodilution.80 Still, TDCO devices require the placement of a central venous catheter, as well as catheterization of a large artery, therefore, there is intense interest in the development of CO monitors that require either a radial artery catheter (minimally invasive) or no catheterization at all (noninvasive). Although this chapter will review current technologies available at the time of this writing, the pace of change in this particular field is so rapid that the reader would be best served reviewing the latest literature to stay abreast of new developments. The majority of arterial waveform analysis devices, with the exception of FloTrac (Edwards Lifesciences, Irvine, CA), are based on the Windkessel model which was developed by Otto Frank.81 Frank’s model makes three assumptions that allow for mathematical modeling of flow—first, that at steady state, the amount of blood entering and leaving a vessel over one cardiac cycle must be the same (conservation of mass); second, that the vessels downstream of the left ventricle are compliant; and third, that blood flow travels in one direction. The simplest mathematical model contains only two elements—a resistor and a capacitor, but models, including additional elements such as aortic input impedance and inductance, have been proposed.82 For additional details on the mathematical features of the Windkessel model, see more detailed reviews on the topic83 (Fig. 12.8).
Hemodynamic Monitoring in Thoracic Surgical Patients
Abstract
Keywords
Introduction
Blood Pressure Monitoring
Oscillometry
Tonometry
Volume Clamp Technique
Intraarterial Catheterization
Central Venous Pressure
Pulmonary Artery Pressure
Cardiac Output Monitoring
Pulmonary Artery and Transpulmonary Thermodilution
Arterial Waveform Analysis
Full access? Get Clinical Tree
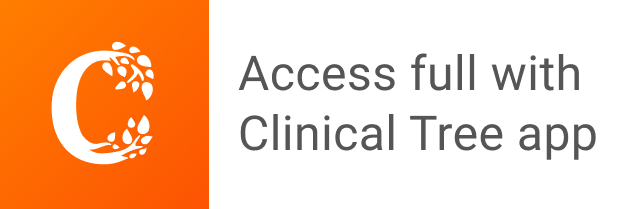