Hemo stasis and Transfusion Medicine
Louanne M. Carabini
Glenn Ramsey
Key Points

































Related Matter
Cerebral Aneurysm Coiling
Formation and Lysis of Fibrin
Introduction

This chapter begins with a review of primary and secondary hemostasis, fibrinolysis, and regulation of the coagulation pathway. We continue with a description of the most common coagulation profile tests followed by the method for blood product collection and storage. The therapeutic indications and risks associated with blood component therapy are discussed at length. The chapter also includes extensive clinical sections discussing congenital and acquired deficiencies in hemostasis and coagulation, as well as an up-to-date presentation of available pharmacologic treatment medications to maintain a balanced hemostatic mechanism.
Hemostasis and Coagulation
Primary Hemostasis

Platelets adhere to sites of endothelial disruption, undergo activation to recruit more platelets and amplify the platelet response, and then cross-link with fibrin, the end product of the plasma clotting factor cascade, to form a platelet plug. Primary hemostasis (Fig. 16-1) describes the initiation of the platelet plug and clotting mechanism.
Adherence
When the endothelial lining is disrupted to expose the underlying matrix, platelets attach to collagen via surface integrin receptors—glycoproteins
(GP) Ia/IIa and GP VI (Fig. 16-1A). Collagen adherence is favored in low-shear conditions such as venous circulation.2 In high-shear arterial blood flow, von Willebrand factor (vWF) from endothelial cells and from preexisting clot binds to integrin Ib/IX, the other major adherence anchor.3 In capillary blood flow, platelets are pushed to the periphery by RBCs, so anemia lessens platelet contact and reduces platelet function.2
(GP) Ia/IIa and GP VI (Fig. 16-1A). Collagen adherence is favored in low-shear conditions such as venous circulation.2 In high-shear arterial blood flow, von Willebrand factor (vWF) from endothelial cells and from preexisting clot binds to integrin Ib/IX, the other major adherence anchor.3 In capillary blood flow, platelets are pushed to the periphery by RBCs, so anemia lessens platelet contact and reduces platelet function.2
Activation
Platelet activation can be mediated by numerous signaling pathways from the platelet surface (Fig. 16-1B). In “outside-in” signaling, a central target is phospholipase C (PLC). The above adherence integrins trigger pathways to activate PLC.2 Another set of surface receptors, G-protein–coupled receptors (GPCRs), are activated by an array of corresponding agonists, including thrombin from the factor clotting cascade, adenosine diphosphate (ADP), thromboxane A2 (TxA2), serotonin, epinephrine, and vasopressin. Each of these agonist–GPCR pairings set off activation pathways for PLC as well.4
Activated PLC leads to several structural changes in the platelets. Via inositol-1,4,5-triphosphate (IP3), calcium (Ca2+) is released from storage tubules. Calcium ions catalyze release of dense granules and α-granules at the platelet surface. These granules contain ADP, serotonin, and more Ca2+, all of which can activate more platelets.2 α-granules contain numerous proteins, including factor V, fibrinogen, and platelet factor 4 (PF-4), which promotes clotting by binding and neutralizing heparin-like
compounds and heparin.2 (This heparin–PF-4 complex is the target antigen for the antibodies causing heparin-induced thrombocytopenia [HIT], discussed in depth later in this chapter.) Calcium also facilitates rearrangement of the platelet microskeleton to change the platelet shape from round and discoid to flat and spiky. Furthermore, the Ca2+ helps activate phospholipase A2 (PLA2), which releases arachidonic acid (AA) from the platelet membrane. AA, as catalyzed by cyclooxygenase-1 (COX-1), is modified to TxA2, which can then activate more platelets.3 Activated platelets also have surface P-selectin and surface-bound and released CD40 ligand, and they release circulating microparticles and attract and activate leukocytes; these features further contribute to hemostasis and also play a role in inflammation.2
compounds and heparin.2 (This heparin–PF-4 complex is the target antigen for the antibodies causing heparin-induced thrombocytopenia [HIT], discussed in depth later in this chapter.) Calcium also facilitates rearrangement of the platelet microskeleton to change the platelet shape from round and discoid to flat and spiky. Furthermore, the Ca2+ helps activate phospholipase A2 (PLA2), which releases arachidonic acid (AA) from the platelet membrane. AA, as catalyzed by cyclooxygenase-1 (COX-1), is modified to TxA2, which can then activate more platelets.3 Activated platelets also have surface P-selectin and surface-bound and released CD40 ligand, and they release circulating microparticles and attract and activate leukocytes; these features further contribute to hemostasis and also play a role in inflammation.2
Stabilization
The activated PLC initiates “inside-out” signaling of GP IIb/IIIa via diacylglycerol (DAG) and protein kinase C (Fig. 16-1C). This changes the shape of GP IIb/IIIa, which permits it to better bind fibrin and vWF. These proteins can bridge to other activated platelets.3 The fibrin binding can also enmesh the platelets, contributing to the formation of the platelet plug in the convergence of the platelet and clotting factor systems.
Inhibition
To maintain hemostatic balance, platelets are naturally inhibited in their endothelial environment. Endothelial cells secrete prostaglandin I2 (PGI2), which binds to a surface receptor to signal increased cyclic adenosine monophosphate (cAMP). Elevated cAMP activates protein kinase A (PKA), a multisite inhibitor of vWF adherence, TxA2 activation, and PLC internal signaling. However, cAMP is metabolized by cAMP phosphodiesterase (PDE). Endothelial cells also secrete nitric oxide (NO), which at high levels initiates a signaling pathway leading to inhibition of the TxA2 receptor.4
Mechanisms of Antiplatelet Medications

Secondary Hemostasis

The extrinsic pathway (Fig. 16-2A–E). The process begins when endothelial disruption exposes tissue factor (TF) on underlying cell membranes (A), extrinsic to the circulation—hence
the term “extrinsic pathway.” TF binds both VII and VIIa, which circulates at low levels, and is a cofactor for the activation of factor VII (B). VIIa enzyme, TF cofactor, cell membrane phospholipid, and Ca2+ form the first complex, a low-efficiency extrinsic-pathway “tenase” which activates factor X and factor IX (C). Then Xa enzyme, its cofactor Va (derived in large part from factor V released from activated platelet α-granules), phospholipid, and Ca2+ assemble to form the second complex, a “prothrombinase,” which converts prothrombin (II) to thrombin (IIa) (E).5
the term “extrinsic pathway.” TF binds both VII and VIIa, which circulates at low levels, and is a cofactor for the activation of factor VII (B). VIIa enzyme, TF cofactor, cell membrane phospholipid, and Ca2+ form the first complex, a low-efficiency extrinsic-pathway “tenase” which activates factor X and factor IX (C). Then Xa enzyme, its cofactor Va (derived in large part from factor V released from activated platelet α-granules), phospholipid, and Ca2+ assemble to form the second complex, a “prothrombinase,” which converts prothrombin (II) to thrombin (IIa) (E).5
The intrinsic pathway (Fig. 16-2F–J). Thrombin has several central functions. It activates platelets via surface receptors PAR-1 and PAR-4 (see Primary Hemostasis), cleaves more V to Va (F), and initiates the “intrinsic” (intravascular) coagulation pathway by cleaving factor XI to XIa (G). XIa cleaves more IX to IXa (H). Thrombin also activates VIII to VIIIa (G). (VIII is carried and stabilized in the plasma by vWF until needed, and vWF deficiency results in low plasma VIII levels also.) The third complex is then formed: IXa enzyme, VIIIa cofactor, phospholipid, and Ca2+. This is a high-efficiency intrinsic-pathway “tenase” (I) which provides many times more Xa for more prothrombinase complex (J). Ultimately, thrombin cleaves fibrinogen to fibrin monomers, which then polymerize extensively. Fibrin polymers are cross-linked by factor XIIIa (also activated by thrombin) to form the stable fibrin clot (K). Fibrin also cross-links activated platelets by their GP IIb/IIIa receptors to enmesh platelets and fibrin in the platelet plug (see Primary Hemostasis).5
All of these clotting factors are primarily produced in the liver, except for VIII, which is also released by endothelial cells and is well maintained in liver disease. The plasma half-life of most clotting factors is around 1.5 to 3 days, but those of the initiating factor VII (6 hours) and the cofactors V and VIII (8 to 12 hours) are much shorter. Four critical enzyme factors—VII in the extrinsic tenase, IX in the intrinsic tenase, X in the prothrombinase, and prothrombin (II)—must be carboxylated at multiple glutamic acid residues after translation, in order to interact with phospholipid and Ca2+. Vitamin K in its reduced form is the cofactor for the glutamyl-carboxylase enzyme, and thus these factors are vitamin K–dependent.5
Inhibition of Clotting Factors
The clotting pathways have three main regulatory inhibitors: (1) TF pathway inhibitor (TFPI), (2) antithrombin-III (AT-III), and (3) protein C and its activation by the “protein C-ase” complex (Fig. 16-3).1,2,3,4,5 (1) TFPI inhibits the external tenase complex by binding to the VIIa protease and to its Xa product. TFPI is produced in endothelial cells, from which its release is stimulated by heparin. Heparin in turn binds to and raises the inhibitory efficiency of TFPI. (2) AT-III is a serine protease inhibitor, or serpin. Serpins disrupt the active sites and increase the clearance of their target proteases. AT-III inhibits proteases in all clotting pathways: VIIa in intrinsic tenase, Xa in prothrombinase, XIa and IXa in the intrinsic tenase pathway, and thrombin. AT-III’s inhibitory function is greatly increased when bound to heparin. (3) Protein C-ase is an enzymatic complex with the same structure as the coagulation complexes above: An enzyme, thrombin, its cofactor thrombomodulin, phospholipid, and Ca2+. Thrombomodulin is expressed on endothelial cell membranes. In the protein C-ase complex, thrombin cleaves and activates protein C. Activated protein C (APC) brakes clotting by cleaving VIIIa and Va, the cofactors for the external tenase and the prothrombinase complexes. Protein C has a short half-life of 6 hours. Protein S is thought to be a cofactor for protein C; both are vitamin K–dependent.5
Fibrinolysis

The major activator of plasminogen in the blood is tissue plasminogen activator (tPA), which is secreted from endothelial cells. When associated with cross-linked fibrin, tPA becomes much more efficient. Once some plasmin is formed, it cleaves tPA to a more active form. tPA also directly cleaves fibrin polymers. In tissues, urokinase plasminogen activator is important in fibrinolysis. Urokinase is secreted from the endothelial cells and the kidney. Plasmin also activates urokinase to a more active form.
Inhibition of Fibrinolysis

activated by the thrombin–thrombomodulin protein C-ase complex. TAFI cleaves fibrin and fibrin polymers in a fashion which inhibits the action of tPA, and TAFI also inhibits the action of plasmin on fibrin. α2-antiplasmin binds to plasmin and blocks its action, although this also slows the metabolism of plasmin.
Laboratory Evaluation of Hemostasis

Laboratory Evaluation of Primary Hemostasis
The normal automated platelet count in adults is approximately 150,000 to 400,000/μL. The peripheral blood smear should be examined in specimens with abnormal platelet counts or size. Microscopic review may reveal clotted specimens, artifactual platelet clumping in vitro, or abnormal platelet morphology. Large platelets are seen in some congenital disorders. One of the first platelet function tests (PFTs) was the template bleeding time, in which a standardized small cut is made on the subject’s forearm and the bleeding duration timed. However, this test is invasive, labor-intensive, impractical to repeat frequently, poorly reproducible, and only modestly predictive for bleeding problems.
In vitro PFTs use various platelet agonists to activate and aggregate the patient’s platelets.7 For example, the PFA-100® device (Siemens, Munich, Germany) simulates capillary blood flow through a chamber after activation by collagen and either epinephrine or ADP. Prolonged “closure time” with collagen/epinephrine but not collagen/ADP suggests aspirin or other antiplatelet medications, whereas when both pairs are abnormal, other congenital or acquired platelet dysfunctions may be present. This type of testing is sometimes used as a screen in patients with a history suggesting platelet problems or von Willebrand disease (vWD). However, the sensitivity and specificity are low. False negatives are common, and abnormal results can also be caused by thrombocytopenia, uremia, or anemia. Several other devices test for specific antiplatelet medication effects from aspirin or P2Y12 inhibitors.7

vWD is a factor deficiency which imparts clinical features of platelet dysfunction, due to the central role of vWF in cross-linking activated platelets to form the platelet plug.9 Up to 1% of all patients have vWD, with a wide range of severity due to either quantitative or functional defects of vWF. Diagnostic testing is integral to deciphering the specific defect and type of vWD to ensure the appropriate treatment. Since vWF is the carrier for factor VIII (FVIII) in plasma, vWF protein levels usually correlate with FVIII levels. Initial testing for vWD should include the vWF antigen level, vWF activity level, and FVIII activity level for comparison with vWF. Blood group O persons have shorter plasma half-life and lower normal levels of vWF, so ABO blood typing may be needed to interpret a borderline vWF level. Type 1 vWD is a quantitative deficiency, with decreased antigen and activity. Type 2 vWD may have normal antigen levels, but decreased activity from a defective protein. Within type 2, there are several subtypes with different molecular defects, and specialized identification is needed in order to determine the best therapy. Type 3 vWD is a rare, very severe autosomal recessive deficiency.9 The clinical features and management of vWD are discussed later in this chapter.
Laboratory Evaluation of Secondary Hemostasis and Coagulation

until complete fibrin clotting is observed. Representative normal ranges are around 12 to 15 seconds for the PT and 25 to 35 seconds for the aPTT, but are defined by each laboratory using its own equipment, reagents, and normal specimens. Testing is routinely performed at 37°C, but hypothermia in the patient impairs the enzymatic reactions of clot formation.
Clotting physiology is more complicated than the traditional diagrams of separate cascade pathways for these two tests. We have seen that thrombin from the intrinsic pathway activates the extrinsic pathway. In vitro, the aPTT clotting test is activated by synthetic contact materials which initiate via factor XII, so deficiencies of XII and other related contact factors cause a prolonged aPTT. However, deficiencies of these contact factors do not cause bleeding and may in fact be associated with impaired fibrinolysis and thrombosis. Fibrinogen activity is also a critical parameter. Most assays measure the functional conversion of fibrinogen to fibrin, although the fibrinogen protein level can also be measured for comparison to assess fibrinogen dysfunction. Normal fibrinogen levels are around 150 to 400 mg/dL.
Mixing Studies
To investigate unexpectedly elevated PT or aPTT values, the test should be repeated after mixing the patient’s plasma with equal volumes of normal plasma. Even in severe factor deficiencies, the PT or aPTT shows substantial correction toward normal in a mixing study. However, if the patient’s plasma contains an inhibitor or an anticoagulant, the normal plasma will also be affected and the PT or aPTT will not be correct.
Individual factor level activities are determined by the degree of correction which patient plasma gives when mixed with factor-deficient plasma. The classic congenital factor deficiencies are FVIII deficiency (hemophilia A) and factor IX deficiency (hemophilia B). Both are X-linked and thus nearly always in males. Factor XI deficiency is most often seen in persons of Ashkenazi Jewish descent. Acquired factor deficiencies usually involve multiple factors.10 The vitamin K–dependent factors are II (thrombin), VII, IX, and X. In liver disease, all factor synthesis is deficient except FVIII, which also comes from endothelium. However, FVIII and other factors can be low in disseminated intravascular coagulation (DIC). As noted, FVIII may be low as part of vWD. Isolated factor X deficiency occurs in some patients with amyloidosis because the abnormal protein absorbs this factor. Performing a set of factors V, VII, and VIII may suggest a pattern to aid in the diagnosis of specific clinical syndromes.
Coagulation inhibitors are substances, usually antibodies, which block one or more clotting factors. Most do not cause bleeding; the most common examples are lupus anticoagulants (LAs), one type of antiphospholipid antibodies (APLA) discussed below in thrombosis tests. However, factor-specific inhibitor antibodies can block clotting in vivo and cause bleeding.10 They are identified by their effect on the plasma factor’s activity and semiquantified by assessing how much interference the patient’s plasma gives to factor level measurements in normal plasma. Some severe hemophiliacs and other factor-deficient patients develop alloantibodies to therapeutic clotting factors, interfering with treatment and necessitating alternative factor therapies or immunosuppression. Bovine thrombin used for topical hemostasis can induce cross-reacting antibodies to the patient’s own factor V. Autoantibodies to specific clotting factors, most commonly FVIII, can cause serious coagulopathy.

Three other tests are commonly performed during surgery with whole-blood specimens: The activated clotting time (ACT), ecarin clotting time (ECT), and viscoelastic whole-blood clotting with thromboelastography. The ACT, a point of care test, assesses the intrinsic clotting pathway and is used mainly to monitor heparin anticoagulation and its protamine reversal during cardiopulmonary bypass or vascular surgery. The ECT also describes the intrinsic clotting function, but it is primarily used for measuring the clinical effects of direct thrombin inhibitors (DTIs) such as bivalirudin. These agents are often used for patients with HIT. The ACT and aPTT also reflect the clinical efficacy of DTIs, but at high doses required for cardiopulmonary bypass, ECT is more accurate.11
Thromboelastography
Whole-blood clotting and fibrinolysis can be assessed by viscoelastic testing in thromboelastography (TEG®, Haemoscope Corp., Niles, IL, USA) or rotation thromboelastometry (ROTEM®, Pentapharm GmBH, Munich, Germany). These tests measure the rate, strength, and lysis, if any, of clot formation. Numerous parameters can be measured with these tests; accordingly the TEG–ROTEM working group attempted to standardize the parameters obtained from both testing modalities in order to make them more clinically relevant. There are minor differences in the mechanisms for TEG versus ROTEM; however, both involve the use of whole blood in a heated cup with the addition of a sensor pin. The cup or the pin oscillates while the blood clots. The increasing resistance to oscillation is transmitted through the sensor pin and a graphic depiction of clot formation is displayed in the thromboelastogram.12 The patterns obtained can implicate defects in factor levels, platelet function, fibrinogen concentration, and the presence of abnormal fibrinolysis, the latter which is difficult to measure rapidly otherwise. Testing can be performed in the presence of inhibitors of heparin or fibrinolysis to help judge whether these drugs would be effective. This test format has also been adapted to assess antiplatelet therapy in patients with ventricular assist devices.13 Thromboelastography is helpful in determining the appropriate therapy, including platelets, plasma, fibrinogen replacement, or antifibrinolytics, as complex bleeding syndromes such as massive hemorrhage with consumptive or dilutional coagulopathy progress.
Diagnosis of Thromboembolic Disorders

or acquired predisposing abnormality which tips the hemostatic systems toward thrombosis. Discovery of one or more risk factors may influence the course of therapy and suggest benefit from family studies.
Congenital Risk Factors for Thrombosis
The most commonly tested congenital problems discussed below increase the risk of VTE.14 Although arterial thrombosis may involve a few of these factors, platelets are more directly involved on the arterial side, and congenital contributions are less well defined. Some investigators have described a “sticky platelet syndrome,” with hyperactive platelet aggregometry. Although severe congenital problems may present in childhood, they are rare; most thrombotic presentations are in adulthood over a lifetime of potential risk. Congenital problems are mostly categorized as deficiencies in antithrombotic pathways or hypercoagulable clotting factors.
Several congenital factors involve the protein C-ase complex and its function. The most common hypercoagulable mutation is factor V Leiden (FVL), in about 5% of Caucasians.15 FV is the cofactor for factor X when the latter activates prothrombin to thrombin. APC is the natural brake on FV, by cleaving it at Arg506. FVL carries the autosomal dominant mutation Arg506Gln, rendering FV fairly resistant to APC. Thus FV is overactive and thrombin formation is favored. The FVL polymorphism is readily identified genetically. However, a small percentage of persons with resistance to APC have other mutations in FV or other conditions. Therefore, slightly more inclusive is the functional clotting test for APC resistance, which assesses plasma clotting time with and without reagent APC.
Protein C itself is functionally deficient in up to 0.5% of the population, with autosomal dominant inheritance. This leads to overactive FVIII and FV cofactors in their respective intrinsic tenase and prothrombinase complexes. Most have low activity and antigen (type I), but some have low activity with normal antigen levels (type II). Homozygous protein C deficiency is a very severe thrombotic disorder beginning in infancy. Protein S deficiency can lead to thrombosis because of its cofactor role to protein C. Around 1 in 700 persons has autosomal dominant deficiency. Protein S circulates partly bound to the complement C4 binding protein and partly as the unbound (free) active form. Nearly all cases of protein S deficiency can be identified by assaying the free antigen and then categorized as to whether the total antigen is low (type I) or normal (type II). Type II has low function but normal antigen levels, but this is rare. Both protein C and protein S are vitamin K–dependent, and therefore vitamin K deficiency or warfarin interferes with laboratory assessment of their activities. Warfarin-induced skin necrosis in protein C or S deficiencies is discussed in antithrombotic therapy testing below. In AT-III deficiency, the relative lack of its normal blocking function on the key enzymes VIIa, IXa, Xa, and XIa leads to thrombosis risk. Testing for AT-III activity will detect both quantitative and qualitative defects.
The best characterized congenital gain in function is the prothrombin mutation G20210A (guanine to adenine). This autosomal dominant condition is found in about 1 in 50 Caucasians, but is much less prevalent in African and Asian backgrounds. Persons with this variant have high circulating prothrombin levels as the reason for thrombosis risk. Genetic testing for the mutation is more definitive than prothrombin levels. Elevated FVIII levels may be a modest risk factor for thrombosis, but FVIII is an acute-phase reactant and rises in many intercurrent conditions. Whether there is an inherited element to persistently elevated FVIII levels is unclear.
Acquired Risk Factors for Thrombosis
Several factors increase the risk of thrombosis.16 APLA are associated with both arterial and venous thrombosis risk. These antibodies bind to phospholipid–protein complexes. Several possible mechanisms for their in vivo effects have been proposed. They may bind to and activate endothelial cells, which in turn could directly tip off coagulation and/or cause vascular injury. They may interfere with phospholipids in the protein C-ase enzyme complex, leading to diminution of protein C’s regulatory function. The various antigenic targets and mechanisms of APLA require multiple tests for their detection. Studies should include tests of clotting function, most notably LA tests, and tests of solid-phase binding to antigen targets, such as anticardiolipin antibodies (ACLA) and anti-β2-glycoprotein-1 (AβGP). AβGP is a protein often present in the phospholipid–protein complex targeted by these antibodies.
LA antibodies are a common cause of prolonged aPTT which does not correct by mixing with normal plasma. It should be emphasized that the prolonged aPTT is a phenomenon of the in vitro test and is NOT associated with bleeding. However, not all LAs prolong the aPTT. Laboratories testing for LA should use at least two different tests to improve detection. One is usually a test based on the aPTT, but modified with phospholipid reagent selected to be sensitive to LA interference. A second clotting-based test is also recommended, such as the dilute Russell viper venom time (DRVVT), in which the snake venom activates factor X in the common pathway, leading to thrombin formation. This test’s reagent phospholipid is adjusted by dilution to be LA-sensitive, but because the venom bypasses the usual initiating factors, the DVVRT is not affected by autoantibody inhibitors of FVIII or other upstream factors, a potential cause of confusion in the PTT-based assays.
ACLA and AβGP antibody tests usually employ enzyme immunoassays (EIA). AβGP may be more specific for physiologic thrombotic effect, by presenting an actual target of in vivo antibodies, whereas ACLA may develop in other conditions such as infections. For example, false-positive non-treponemal syphilis serology is sometimes seen with ACLA.
Hyperhomocysteinemia is a risk factor for venous and possibly arterial thrombosis. This amino acid is made from methionine and is then either converted back or processed to cysteine. The mechanism for thrombosis risk is unclear, but endothelial cell injury has been proposed. Fasting total homocysteine is the initial screening test. Hyperhomocysteinemia can be due to various congenital mutations in homocysteine’s metabolic pathways or can be acquired via vitamin deficiencies affecting its metabolism (folate, B12, B6) or in many other medical conditions.
Monitoring Anticoagulation Therapeutic Agents

Warfarin Anticoagulation
Warfarin therapy must be monitored by the PT and its analogue for this purpose, the international normalized ratio (INR), in order to avoid under- or overcoagulation. PT methods and
reagents can widely differ between laboratories, yielding varying PT values for the same degree of factor deficiency. However, each PT test vendor supplies a conversion parameter to express the PT as the INR in patients on warfarin. The INR is a normalized value which is intended to compare results across laboratories for evaluating combined deficiencies of factors II, VII, IX, and X, the warfarin-dependent factors. The INR’s therapeutic range for warfarin anticoagulation is generally 2.0 to 3.0, except for mechanical heart valves and prevention of myocardial infarction (INR 2.5 to 3.5).17
reagents can widely differ between laboratories, yielding varying PT values for the same degree of factor deficiency. However, each PT test vendor supplies a conversion parameter to express the PT as the INR in patients on warfarin. The INR is a normalized value which is intended to compare results across laboratories for evaluating combined deficiencies of factors II, VII, IX, and X, the warfarin-dependent factors. The INR’s therapeutic range for warfarin anticoagulation is generally 2.0 to 3.0, except for mechanical heart valves and prevention of myocardial infarction (INR 2.5 to 3.5).17
When warfarin is started or stopped, the factors with the fastest plasma turnover, that is, the shortest half-lives, decline or rise the fastest, respectively. Thus, the inhibitor protein C, with a 6 hr half-life, declines faster than most clotting factors when warfarin takes effect, and this can cause an imbalance toward clotting during the initiation of warfarin therapy. Warfarin-induced skin necrosis is a thrombotic complication often occurring when previously unrecognized congenital protein C deficiency accentuates this imbalance.
Warfarin’s pharmacology is affected by genetic variations in the metabolism of the drug (cytochrome P450, CYP2C9) or its counterbalancing vitamin K (vitamin K epoxide reductase complex subunit 1, VKORC1). Genetic polymorphism testing has been advocated for achieving more rapid therapeutic effect when initiating therapy or in assessing difficulty achieving the target INR, but indications for genetic testing are still under investigation. The INR is not calibrated to evaluate non-warfarin deficiencies such as liver disease, which affects most other clotting factors, and thus strictly speaking, the INR should not be used in other conditions including liver disease.
Heparin Anticoagulation Testing
The aPTT is used to assess heparin anticoagulation. Each laboratory determines its own therapeutic target range for heparin anticoagulation, typically on the order of 1.5 to 2.5 times the normal mean. The laboratory determines the exact range for their test system based on a functional enzymatic test for heparin action, the anti-factor Xa activity (aFXa). (This is a different test than the factor X activity level.) Using the aFXa assay, therapeutic target heparin levels of 0.3 to 0.7 aFXa units/mL are correlated with aPTT results for that range. aFXa testing can be helpful in assessing heparin resistance.
Low-molecular-weight heparin (LMWH) drugs and their analogue, synthetic pentasaccharide (e.g., fondaparinux), do not affect the aPTT assay, and coagulation testing is usually not needed. However, if necessary, the drugs’ plasma activity levels can be assessed by the aFXa assay. This may be helpful in renal failure affecting drug excretion or in pregnant women, obese, and neonates for whom drug levels are less certain after subcutaneous injection. Like heparin, these agents inhibit factor Xa indirectly, that is, via their enhancing effect on antithrombin.
Heparin and to a lesser degree LMWH can stimulate the production of antibodies against the heparin–PF-4 complex. These antibodies can in turn cause HIT and/or activation of platelets to induce thrombosis.18 If thrombocytopenia or thrombosis develops in a patient on these drugs, tests for HIT antibodies are available by EIA or by functional measures such as serotonin release. Patients with HIT must avoid heparin and LMWH.
Several newer anticoagulants have entered clinical practice, as alternatives to heparin in patients with HIT or as alternatives to warfarin. These are direct anticoagulants which are not mediated by antithrombin. The “xaban” class of drugs, including rivaroxaban and apixaban, directly inhibit factor Xa.19 They prolong the PT and aPTT, but monitoring is not recommended and the INR should not be used. An aFXa-type assay adapted for these drugs is being researched.
DTIs also pose challenges for coagulation testing. These include hirudin from leeches, its recombinant “-rudin” mimicking molecules (bivalirudin, desirudin, lepirudin), and small synthetic molecules acting at the same site as hirudin on thrombin (argatroban, dabigatran). They all prolong the PT and aPTT and interfere with clot-based fibrinogen assays. There is no consensus on how to quantify the effect of these drugs. The ECT has been promulgated.20 Ecarin, an enzyme in snake venom, cleaves prothrombin to a metabolic intermediate which is inhibited by hirudin and its analogues. However, the ECT is not widely available.
Blood Component Production
Blood Collection
The production of blood components is highly controlled by regulations and accreditation requirements in the interests of donor and recipient safety and therapeutic efficacy. Blood donors are carefully screened and tested, and blood products are made in specialized laboratories and other facilities. Regional blood centers collect and provide most blood components for transfusion, although some hospitals collect blood or platelets to augment their supply. Virtually all blood components come from volunteer unpaid donors. Pharmaceutical companies process plasma into various derivatives or synthesize some desired proteins for infusion.
Blood donors undergo a confidential interview to screen for medical problems in their donation safety and for risks of disease transmission to their recipients.21 They are questioned about risk factors, exposure, or signs of human immunodeficiency virus (HIV), hepatitis, and other infections. There are geographically based deferral criteria for tropical exposure to malaria and (in American donors) European exposure to variant Creutzfeldt–Jakob disease (vCJD). The donor’s pulse, blood pressure, and hemoglobin/hematocrit level (US minimum 12.5 g/dL or 38%) are checked. Phlebotomy is performed with validated antiseptic measures to reduce the risk of bacterial contamination in the blood bags. In the United States, donors are deferred for 8 weeks after a whole-blood donation to avoid iron deficiency.
Table 16-1 shows the contents and storage parameters for blood components. In whole-blood donations, 450 to 500 mL of blood is collected into citrate anticoagulant and then separated by centrifugation into RBCs, platelets, and/or plasma. The RBC units usually have most plasma removed and replaced with preservative. In the United States, the plasma must be frozen within 6 hours of collection to be labeled fresh frozen plasma (FFP). A large proportion of plasma is now made as plasma frozen within 24 hours, with minimal effect on clotting factor content compared to FFP. Cryoprecipitate is made from barely thawed FFP, which has a precipitate enriched in fibrinogen; the precipitate is isolated by centrifugation and refrozen.22 Five bags of “cryo” comprise a typical adult dose. Whole-blood–derived platelets (sometimes called “random-donor platelets”) are derived from platelet-rich plasma in the United States and from the buffy-coat centrifugation layer in other countries.23 Four to six units are pooled to yield one adult-sized dose of platelets. Traditionally, pooling was done at the hospital just before transfusion, but blood centers can now provide pre-pooled platelets to hospitals.
Blood components are also collected by apheresis, in which the donor’s blood is processed by ex vivo centrifugation, the
desired component(s) are siphoned off into citrate anticoagulant, and the rest returned to the donor. Most platelets are produced by apheresis (sometimes called single-donor platelets). Plasma and RBCs can also be collected by apheresis, and if the donor’s blood volume and cell counts permit, two doses of the desired component can be obtained in one collection session.
desired component(s) are siphoned off into citrate anticoagulant, and the rest returned to the donor. Most platelets are produced by apheresis (sometimes called single-donor platelets). Plasma and RBCs can also be collected by apheresis, and if the donor’s blood volume and cell counts permit, two doses of the desired component can be obtained in one collection session.
Table 16-1. Blood Components | ||||||||||||||||||||||||
---|---|---|---|---|---|---|---|---|---|---|---|---|---|---|---|---|---|---|---|---|---|---|---|---|
|
All donors are checked against files of deferred donors, and all donations are tested for blood-borne infectious agents. Tests for HIV, hepatitis B virus (HBV), and hepatitis C virus (HCV) are universally required.24 The addition of sensitive nucleic acid testing (NAT; e.g., polymerase chain reaction) to routine serology shortens the window period in a recently infected donor down to 7 to 10 days for HIV and HCV and 1 month for HBV. The US FDA also requires testing for syphilis, human T-cell lymphotropic virus (HTLV), and West Nile virus (WNV). Also available for donors at risk is a test for Trypanosoma cruzi (Chagas disease). Cytomegalovirus (CMV) is mainly in WBCs; selected patients at risk for complications of infection from cellular components can receive either CMV-negative units or leukoreduced units.
At some centers, directed donations can be made by family or friends for a specific patient. These donations augment the overall supply of blood and donors, and could reduce the number of donor exposures by using the same donor(s) more than once. However, the infectious risk from these units is not considered any less than from community volunteer donors. Cellular components from blood relatives must be irradiated to prevent the risk of transfusion graft-versus-host disease (GVHD) from closely matched donor lymphocytes which are not rejected by the recipient.
Autologous donations by the patient can be made in advance of scheduled surgery, with physician approval. The usual minimum hemoglobin or hematocrit is 11 g/dL or 33%, respectively, which is lower than the minimum allowed for regular donors. Patients should be on erythropoietin or iron supplementation to support RBC replenishment. In order to achieve a net gain in RBC mass, donations should be scheduled with some lead time within the 6-week storage period to permit erythropoiesis before surgery. Erythropoietin assists with multiple donations. Autologous units are only used for the patient and cannot be given to anyone else, since they often have a lower RBC content than normal requirements, and surgical patients frequently have conditions disqualifying them for regular donation.
Component Processing and Storage

In addition to the routine indications above, LR has been studied for the prevention of transfusion-related acute lung injury (TRALI) and so-called transfusion-related immunomodulation (TRIM), that is, possible immunosuppressive and pro-inflammatory effects mediated by donor WBCs. “Universal” LR of all cellular components is done in many countries, for this and other reasons. However, randomized controlled trials have been mostly inconclusive for benefit. Meta-analysis of available data has highlighted cardiac surgery as a setting in which there may be postoperative survival benefit associated with leukoreduced blood components, based on a small number of studies to date.25
Washing cellular components with saline is mostly done to remove plasma in patients with allergic transfusion reactions such as those who are IgA deficient. It does not affect the antigens on the cells and does not remove enough WBCs to prevent GVHD or HLA alloimmunization.
Irradiation of cellular components is performed to prevent transfusion GVHD from directed-donor units from blood relatives and in highly immunosuppressed patients at risk for this complication: Leukemia, lymphoma, hematopoietic stem cell transplants, congenital cellular immunodeficiencies.26 The units are exposed to gamma irradiation (2,500 cGy) to damage donor WBC DNA and prevent a cellular immune proliferative response to the recipient’s tissues. Irradiation is usually performed in cesium-137 blood irradiators. The blood units do not come in contact with the radioisotope and are not radioactive, but laboratory personnel must comply with radiation safety regulations. Blood units can also be irradiated by cobalt-60 beams in radiation therapy facilities, but in the United States, the FDA regulates this function closely. In recent years, X-ray generators which do not contain radioisotopes have been approved for blood components.
Platelets are stored at room temperature to preserve clotting function, but this increases the risk of bacterial growth in contaminated units, compared to other blood components. Accordingly, bacterial detection has become routine in many countries. Platelet testing must be done after a short period of storage in order to detect growing bacteria. Blood centers can take culture samples from plateletpheresis units and pre-pooled whole-blood–derived platelets before product release. However, culturing is not
feasible from the small volumes of individual whole-blood–derived platelets when the transfusion service is pooling the units just before use. In this situation, bacterial antigen testing is available to transfusion services and is required by accrediting agencies in the United States.
feasible from the small volumes of individual whole-blood–derived platelets when the transfusion service is pooling the units just before use. In this situation, bacterial antigen testing is available to transfusion services and is required by accrediting agencies in the United States.
RBC preservation solutions use CDPA—Citrate for anticoagulation, Phosphate as a buffer, Adenine, and 1 to 2 g of dextrose (D-glucose)—to maintain adenosine triphosphate (ATP) levels and RBC membrane integrity. However, despite preservatives, several metabolic changes occur during RBC storage. 2,3-Diphosphoglycerate (2,3-DPG) is depleted in the first 2 weeks, thus shifting the oxygen dissociation curve to the left and making hemoglobin more avid for O2, although this reverses after transfusion. Furthermore, by the end of the 42-day shelf life of additive-solution RBCs, the pH is 6.5, the plasma K+ is 50 mmol/L from RBC leakage and hemolysis, and 15% to 20% of the RBCs are nonviable.27 RBC storage lesions could be involved in observations linking older units to adverse outcomes such as short-term mortality and multiple organ failure.28 Randomized trials are underway to address this important question.
Plasma Derivatives

Albumin is produced in large quantities for intravascular volume support and is pasteurized at 60°C for sterility. IGs are given for immune support or for immunomodulation to suppress native antibody production. IGs can also withstand robust pathogen inactivation processes, and in some cases are nanofiltered to remove viruses. To be given intravenously, IGs need extra processing to avoid reaction-provoking protein aggregates. Hyperimmune globulins are fractionated from the plasma of donors with high levels of antibody to specific antigens of interest, such as viruses (HBV, CMV, varicella zoster) or the Rh blood group D antigen (RhIG to prevent anti-D formation in RhD-negative women).
Factor concentrates for patients with congenital deficiencies are made with special techniques to ultrapurify factors VIII, IX, XIII, vWF, fibrinogen, and in some countries, factor XI—while also applying pathogen inactivation methods and viral filtration to remove microbes. However, plasma-free recombinant factors VIII and IX are also available to further allay concern about disease transmission, and recombinant activated factor VII (rFVIIa) is approved for rare VII-deficient patients. Some hemophiliacs develop inhibitory antibodies to the FVIII or factor IX they are missing. These inhibitor patients often need products which “bypass” their missing clotting step. The therapeutic potential of prothrombin complex concentrates (PCCs) containing multiple factors, or FVIIa to bypass secondary hemostasis and generate a thrombin burst is discussed later in this chapter. On the antithrombotic side of hemostasis, antithrombin concentrate is available.
Other plasma proteins which are purified for selected deficiencies are complement C1 esterase inhibitor (for hereditary angioedema) and α1-antitrypsin.
Pathogen Inactivation

More robust methods involve agents which damage nucleic acids. When methylene blue, psoralen, or riboflavin is added to the blood bag, they bind to nucleic acids. Then photoactivation is performed with specific wavelengths of light, cross-linking DNA and RNA to prevent microbial function. Not yet approved but under investigation is ultraviolet light treatment of platelets. RBC units are less amenable to photoactivation through the hemoglobin, although riboflavin is being explored. Certain alkylating agents have shown promising microbicidal activity, but modify the RBC membrane enough that subjects have formed antibodies against the treated RBCs.
The adverse effects that limit these techniques include the potential for reduced platelet count increments, alterations in plasma clotting factor levels, and potential toxicity from some of the added agents if they are not sufficiently removed after treatment.
RBC and Platelet Substitutes

Platelets are so complex that it would be difficult to replace their functions fully. However, some early work has emerged on preserved platelets (e.g., freeze-dried or fixed) and on biocompatible platforms bearing hemostatic proteins, such as fibrinogen-coated
albumin beads, which could supply some degree of platelet-like clotting function. Culturing platelets in vitro for therapeutic uses is also being pursued.30
albumin beads, which could supply some degree of platelet-like clotting function. Culturing platelets in vitro for therapeutic uses is also being pursued.30
Blood Products and Transfusion Thresholds
Compatibility Testing

RBC compatibility testing takes 45 to 60 minutes, and much longer if antibodies are found. Hence, testing in advance of scheduled surgery is desirable. In emergencies, uncross-matched group O RBCs can be given, albeit with the risk of non-ABO antibody incompatibility. Group AB is the universal plasma which avoids transfusing anti-A or -B versus the patient’s RBCs.
Red Blood Cells

The original TRICC trial included critically ill but euvolemic patients in a large multicenter randomized control trial. The investigators compared the survival of patients transfused to hemoglobin levels greater than 10 g/dL in the liberal group with patients under a restrictive strategy who were not transfused until their hemoglobin fell to less than 7 g/dL. Overall survival for more than 800 patients did not differ between the groups.32 Subsequent subgroup analysis of the TRICC trial and several meta-analysis and systematic reviews over the past 10 years not only confirmed these results, but established a possible morbidity and mortality risk associated with liberal transfusion management for a variety of different patient populations including those less than 55 years old, trauma patients, and those with stable cardiovascular disease.33,34,35,36
Traditionally, patients with cardiovascular disease and anemia were considered to be at significant risk of tissue ischemia and therefore thought to benefit from higher hemoglobin goals in the perioperative and critical care setting. These recommendations were based on two studies which showed an association between anemia in patients with cardiovascular disease and mortality that improved with transfusion.37,38 Several retrospective studies and systematic reviews have since contradicted these findings by documenting the safety of hematocrits less than 24% during cardiac surgery with cardiopulmonary bypass.34,39 The Transfusion Requirements After Cardiac Surgery (TRACS) trial randomized postcardiac surgery patients to restrictive (hematocrit >24%) versus liberal (hematocrit >30%) transfusion strategies and found no difference in 30-day mortality or severe morbidity. Furthermore, they found that transfusion was an independent risk factor for morbidity and mortality.34,40 The most recent update to the Society of Thoracic Surgeons and the Society of Cardiovascular Anesthesiologists Blood Conservation Clinical Practice Guidelines recognizes that all cardiac surgery patients are at risk for tissue hypoxia and transfusion. These guidelines report that tissue oxygenation does not improve with transfusion for hemoglobin levels greater than 10 g/dL; and they state that “Transfusion is reasonable in most postoperative patients whose hemoglobin is less than 7 g/dL.” For patients with hemoglobin levels between 7 and 10 g/dL the two societies recommend transfusion in patients with “critical noncardiac end-organ ischemia,” active blood loss, or clinical indication of tissue hypoxia (low mixed venous oxygen saturation or electrocardiographic or echocardiographic evidence of myocardial ischemia).41
Patients with cerebrovascular disease or acute neurologic illness such as ischemic stroke, subarachnoid hemorrhage, and traumatic brain injury are at significant risk for secondary injury from tissue hypoxia. There are several studies and reviews devoted to the question of optimal brain tissue oxygen delivery during acute neurologic illness. Acute and chronic anemia initiates physiologic compensatory mechanisms discussed below as well as neuroprotective strategies for tolerating decreases in cerebral oxygen delivery; however, the hemoglobin level at which anemia induces detrimental patient outcomes remains unclear.42 Evidence indicates that a hemoglobin level less than 9 g/dL is independently predictive of poor outcome especially in patients with cerebrovascular injury.43,44 RBC transfusion perioperatively or during critical illness is also independently associated with morbidity and mortality.43,45,46 This leaves the clinician with little guidance to decide when and who should be transfused. Clearly transfused RBCs do not function as well as endogenous erythrocytes and it remains unclear if transfused blood improves overall outcomes.
Physiologic Compensation for Anemia

hypoxia. However, given the ability of the body to compensate for anemia, it is unclear whether increasing hemoglobin in a stable anemic patient actually improves tissue oxygenation. There are several compensatory mechanisms for anemia, most notably (1) increased CO; (2) altered microcirculatory blood flow; and (3) improved tissue oxygen extraction from hemoglobin. These physiologic changes in conjunction with the detrimental impact of RBC storage limit the therapeutic effects of transfusion.48
|
Increased CO. There are several mechanisms for the increase in CO that occurs as compensation for isovolemic hemodilution. First of all, the heart rate increases secondary to a sympathetic surge initiated by anemia and hypoxia.49,50,51 Secondly, higher stroke volume results from increased preload and decreased systemic vascular resistance and afterload. Isovolemic hemodilution occurs when acute blood loss is resuscitated with crystalloid or colloid fluids, thereby maintaining blood volume with lower hemoglobin. This decreases blood viscosity, one component of systemic vascular resistance, and decreases afterload by arterial vasodilation. The arterioles dilate as a result of the release of NO from endothelial cells. Lastly, tissue hypoxia will induce arteriovenous shunting and recruitment of new circulatory beds thereby increasing microcirculatory blood flow.49
Altered Microcirculatory Blood Flow. The decrease in blood viscosity associated with isovolemic hemodilution and chronic anemia improves blood flow especially through the microcirculation where lower shear force rates cause a pronounced vasodilation of capillary beds.47,49,50 This improves venous return by lowering the resistance of venous beds and therefore increases preload and left ventricular filling. Patients with chronic anemia also increase angiogenesis and overall microcirculatory blood volume to compensate for the decreased oxygen-carrying capacity.49 These mechanisms are extremely efficient. In fact, studies in anemic critically ill patients fail to show improvements in tissue oxygenation measures after transfusion.48
Increased Tissue Oxygen Extraction. Anemia causes the oxygen dissociation curve for oxyhemoglobin to shift to the right secondary to increased levels of 2,3-DPG in erythrocytes. This adaptive process is particularly influential for the physiologic compensation of chronic anemia and often the only mechanism necessary to maintain oxygen delivery. Isovolemic hemodilution to hematocrit levels less than 25% generates an increase in 2,3-DPG levels. Furthermore, acidemia associated with acute hemorrhage also shifts the oxygen dissociation curve to the right thereby decreasing the affinity of oxygen for hemoglobin at any partial pressure and improving the tissue oxygen extraction ratio.49,50
There continues to be controversy about RBC transfusion given the lack of evidence to support a universal transfusion threshold. Despite the wealth of literature documenting the potential harm, transfusion continues to be the mainstay of treatment for acute and chronic anemia. Healthy patients are most often able to compensate for anemia and tolerate critically low hemoglobin levels; however, the risk of tissue hypoxia in acute situations or in patients who are unable to compensate such as those with low cardiovascular reserve remains unclear. Each clinician must take into account the patient’s comorbidities, the acuity of anemia, and their ability to compensate adequately without signs or symptoms of tissue hypoxia (Table 16-3).52 Furthermore, it is important to measure the response to transfusion with follow-up hemoglobin levels in order to aid in the diagnosis of consumptive anemia, hemolysis, or ongoing bleeding as well as to guide further transfusions. In stable patients without ongoing bleeding, the hemoglobin should rise 1 g/dL with approximately 3% rise in hematocrit for each unit of packed RBCs given.52
The above discussion focuses on isovolemic hemodilution secondary to chronic anemia or acute hemorrhage post fluid resuscitation when compensatory mechanisms have had time to balance the detrimental effects of decreased hemoglobin levels. It is important to recognize that transfusion may be necessary
prior to the availability of measured hemoglobin and hematocrit levels such as during acute hemorrhage, intraoperative bleeding, or trauma resuscitation. In the case of uncompensated blood loss, hemoglobin levels may be normal or misleadingly high. In these situations, the clinician must estimate blood loss from the patient’s hemodynamic picture and assessment of the operative field in order to guide their transfusion management. Recent recommendations for intraoperative transfusion during massive bleeding focus on patients with class II hemorrhage (blood loss of 15% to 30% estimated blood volume) with preexisting anemia or cardiovascular disease and all patients with class III to IV hemorrhagic shock (blood loss of 30% to 40% estimated blood volume).53 In these cases, the British Committee for Standards in Haematology guidelines for the management of massive transfusion recommend a goal hemoglobin over 8 g/dL.54
prior to the availability of measured hemoglobin and hematocrit levels such as during acute hemorrhage, intraoperative bleeding, or trauma resuscitation. In the case of uncompensated blood loss, hemoglobin levels may be normal or misleadingly high. In these situations, the clinician must estimate blood loss from the patient’s hemodynamic picture and assessment of the operative field in order to guide their transfusion management. Recent recommendations for intraoperative transfusion during massive bleeding focus on patients with class II hemorrhage (blood loss of 15% to 30% estimated blood volume) with preexisting anemia or cardiovascular disease and all patients with class III to IV hemorrhagic shock (blood loss of 30% to 40% estimated blood volume).53 In these cases, the British Committee for Standards in Haematology guidelines for the management of massive transfusion recommend a goal hemoglobin over 8 g/dL.54
|
Platelets

Transfusion is not necessary for platelet counts >100,000/μL in clinically stable patients without suspicion of platelet dysfunction. Most patients with counts <50,000/μL and clinical bleeding require therapeutic transfusion.31,55,56 There are several relative indications for transfusion of platelet counts between 50,000 and 100,000/μL. In particular, patients having surgery on vital organs such as the eye or central nervous system, or patients with multiple traumatic injuries, benefit from higher transfusion goals.53,55,56 Furthermore, patients undergoing massive transfusion or hemorrhage with estimated blood loss of more than two blood volumes and ongoing bleeding should have a transfusion threshold of at least 75,000/μL to ensure the level does not fall below 50,000/μL.53,54
|
|
Platelet transfusion should not be guided by platelet counts alone, but also by the clinical suspicion of platelet dysfunction which can be inherited or acquired. Qualitative dysfunction is often associated with systemic diseases such as uremia, liver failure, and disseminated intravascular coagulopathy. It also occurs after cardiopulmonary bypass, extracorporeal circulation such as dialysis or plasmaphoresis, and as a result of medication side effects59 (Table 16-5). Regardless of the platelet count, if bleeding is out of proportion to the level of thrombocytopenia, qualitative deficiency should be suspected and treated.53,56
The average dose of platelets is one concentrate from apheresis donation or pools of five to eight concentrates from whole-blood or buffy-coat collections. These “units” generally contain about 3 × 1011 to 4 × 1011 platelets each.53,55,56 Once platelets are given, a posttransfusion response should be followed to guide further therapy and to rule out ongoing consumptive pathophysiology. Refractoriness at 20 to 24 hours is generally associated with older platelets or increased consumption secondary to fever, infection, bleeding, or medications.56 Adjunctive therapy for platelet dysfunction can be established with antifibrinolytics, DDAVP (1-deamino-8-D-arginine vasopressin, desmopressin), PCCs, or rFVIIa as discussed later in this chapter.55,58
Fresh Frozen Plasma
FFP is a blood component that contains all the factors involved in hemostasis. (As used here, “FFP” also includes plasma frozen within 24 hours.) The preparation and storage method discussed previously in this chapter involves either separation from whole
blood or apheresis; both maintain normal levels of stable factors and at least 70% normal levels of labile factors such as FVIII and FV. Prior to use, each unit must be thawed at 30 to 37°C, but can be stored at 4 ± 2°C for up to 24 hours as thawed FFP or 5 days as thawed plasma.53,56 FFP must be ABO-compatible with the recipient to avoid transfusion of donor anti-A and anti-B antibodies that may lead to hemolysis.60
blood or apheresis; both maintain normal levels of stable factors and at least 70% normal levels of labile factors such as FVIII and FV. Prior to use, each unit must be thawed at 30 to 37°C, but can be stored at 4 ± 2°C for up to 24 hours as thawed FFP or 5 days as thawed plasma.53,56 FFP must be ABO-compatible with the recipient to avoid transfusion of donor anti-A and anti-B antibodies that may lead to hemolysis.60
|
FFP is indicated for the treatment of coagulopathy secondary to congenital or acquired factor deficiencies. The specific indications for FFP outlined in Table 16-6 are based on a compilation of guidelines from the ASA, the British Committee for Standards in Haematology, and the Italian Society of Transfusion Medicine and Immunohaematology (SIMTI).31,53,56,60,61 The initial therapeutic dose of FFP averages 10 to 15 mL/kg in an attempt to obtain at least 30% factor activity. Repeat dosing should follow the results of serial diagnostic coagulation tests such as the PT and aPTT.31,53,56,60,61 Although the guidelines for FFP continue to recommend prophylactic transfusions in patients at risk for bleeding, there is no evidence to support the efficacy of FFP reducing red cell transfusion, morbidity, or mortality, especially when given to patients with mild derangements in PT or aPTT and no clinical signs of bleeding.61,62 The transfusion of plasma carries risks of several transfusion reactions, most notably TRALI, allergic reactions, and cardiopulmonary overload. The burden of adverse reactions in conjunction with limited prophylactic benefits and high frequency of inappropriate use makes FFP arguably the riskiest blood component transfused.60,61,63,64
Over the past decade there has been growing debate over formula-driven resuscitation protocols for trauma patients with major bleeding. Traditionally trauma patients were resuscitated first with fluid and red cell units only to receive FFP based on the results of coagulation tests.54 This method perpetuates dilutional coagulopathy resulting in prolonged microvascular bleeding.65 More recent studies show improved outcomes with higher ratios of red cell units to FFP (more than 3:2).65,66 However, these findings are mostly retrospective and likely confounded by injury severity and the potential “survivor bias” that occurs when patients die of massive hemorrhage before FFP can be thawed and transfused.66,67 The optimal management strategy for immediate resuscitation of trauma patients and those with acute massive hemorrhage is still under investigation. However, it is clear that coagulopathy driven by ongoing bleeding requires rapid response, and the best treatment strategies involve active management protocols to maintain intravascular volume, avoid platelet dysfunction, and facilitate hemoglobin and factor replacement.
Cryoprecipitate

Fibrinogen is primarily responsible for clot stabilization, but it also assists with platelet activation and aggregation and, at adequate concentrations, may compensate for low thrombin states.69 Traditionally, the threshold for fibrinogen replacement has been levels less than 80 to 100 mg/dL.31,60,68 However, a few studies of acquired hypofibrinogenemia with massive bleeding document the need for higher levels (150 to 200 mg/dL) to optimize clot stabilization.70,71 A single dose of five pooled bags typically raises the fibrinogen concentration by 50 mg/dL.68
|
The Risks of Blood Product Administration

Infectious Risks of Blood Product Administration
Since the introduction of NAT for the major transfusion-transmittable viral infections (HCV in 2000, HIV in 2003, and HBV in 2006) the residual risk of infection from blood product transfusion has decreased substantially.72 Prior to the institution of NAT, the blood supply was simply tested for the presence of viral antibodies leaving a long window period when the blood is infectious, but there was not sufficient time for the donor to mount a large enough antibody response to be detected by conventional testing. NAT increased the sensitivity of donor screening by testing for the presence of specific viral DNA. This significantly shortens the window period between when a donor gets infected and when the viral load is detectable. The residual risk of transfusion-transmitted infections depends mostly on the relative length of this window period determined by the reproductive rate of each virus and the prevalence of the disease. There is an additional risk of false negatives or mistaken release of quarantined blood products; however, these events account for less than 0.5% of the residual risk of transfusion-transmitted viral infection.73
Table 16-8. Residual Risk of Transfusion-Transmitted Infections | ||||||||||||||||||||||||||||||||||||||||||||||||||||||
---|---|---|---|---|---|---|---|---|---|---|---|---|---|---|---|---|---|---|---|---|---|---|---|---|---|---|---|---|---|---|---|---|---|---|---|---|---|---|---|---|---|---|---|---|---|---|---|---|---|---|---|---|---|---|
|
The true risk of transfusion-transmittable infections is difficult if not impossible to accurately quantify given the variability of donor response to inoculation and the immune state of the recipient. However, the residual risk can be mathematically modeled from the prevalence of infection in donors and the known window period of each viral illness. The estimated residual risk of major viral infection and the viral-specific window periods are shown in Table 16-8 based on the reported incidence of infection in donors for the American Red Cross Blood Services.73 These residual risks are likely overestimates since not all transfusions of known infected blood products result in recipient infection.74,75 The mathematical modeling of residual risk assumes 100% infectivity with even one infectious unit of viral particles per blood bag. It also presumes that the collection and storage process is harmless to viral reproduction. Furthermore, viral infectivity will vary depending on the acute phase of infection when an immunocompetent recipient could resist transmission, or the chronic phase of infection when antibodies are present and reduce infectivity.75
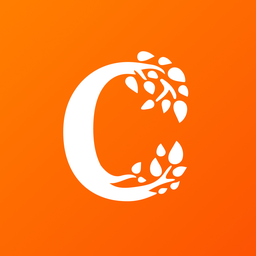
Full access? Get Clinical Tree
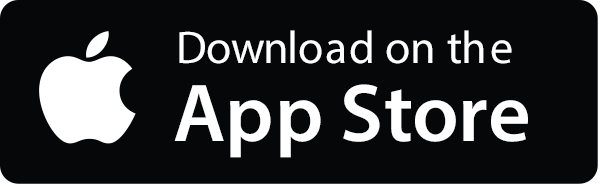
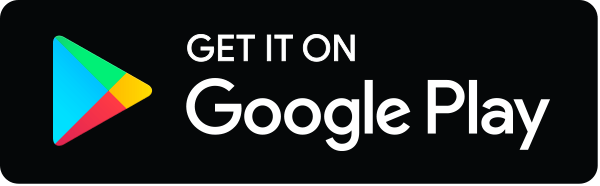