Larynx
The larynx is a complex structure that lies anterior to the 4th to the 6th cervical vertebra and consists of several muscles, their ligaments, and associated cartilaginous structures (Fig. 24-2). The inlet of the larynx is bordered by the epiglottis, aryepiglottic folds, and the arytenoids. The larynx itself bulges into the pharynx posteriorly creating a deep pharyngeal recess anterolaterally on either side, the pyriform fossae. The pyriform fossae, which lie to each side of midline, are clinically relevant because of their tendency to trap food or foreign objects in the pharynx and as potential sites for the application of topical anesthesia to block the internal branch of the superior laryngeal nerve. The larynx serves as the organ of phonation, plays an important role in coughing, and in protection of the airway from entrainment of solids and liquids during swallowing.5

The primary support structure of the larynx is the thyroid cartilage that forms the point of articulation of the paired arytenoid cartilages with the vocal ligaments and their controlling musculature. Other essential structures include the hyoid bone and its attachments, the epiglottis, the cricoid cartilage, and the corniculate cartilages. The hyoid bone is a U-shaped bone that is attached directly to the stylohyoid ligament and muscle, to the mandible and tongue by the hyoglossus, mylohyoid, geniohyoid, and digastric muscles, and to the pharynx by the middle pharyngeal constrictor muscle. Beneath the hyoid bone is the remainder of the larynx suspended by its attachment, the thyrohyoid membrane and muscle. Although its function other than as a flexible anchor is unclear, it is possible to bisect its mandibular attachments (“suprahyoid release”) and mobilize the larynx in order to facilitate its caudal displacement in tracheal resection procedures. The epiglottis is the midline elastic cartilage found inferior to the base of the tongue. It is anchored anteriorly to the hyoid bone and inferiorly to the inside of the anterior portion of the thyroid cartilage immediately above the vocal cords. Bilateral folds of the epiglottis curve posteriorly to form a mucosal ridge attached to the arytenoid cartilages sitting on top of the posterior lamina of the cricoid, the aryepiglottic folds. The epiglottis, aryepiglottic folds, and the corniculate tubercles form the inlet into the glottis below. The thyroid cartilage contains the larynx with its paired lamina fused anteriorly at the laryngeal prominence and extending posteriorly to terminate in superior and inferior horns or cornu. The thyroid cartilage serves as a stable point of attachment for numerous small muscles and ligaments which manipulate the vocal cords. The thyroid cartilage also has a mobile, membranous attachment to the cricoid ring.
The paired vocal cords attach posteriorly to the vocal process of each arytenoid and anteriorly meet at the junction of the thyroepiglottic ligament of the anterior portion of the thyroid cartilage. The triangular opening formed by the vocal ligaments is the glottis with its apex anteriorly (Fig. 24-3). The mean length of the relaxed open glottis is approximately 23 mm in males and 17 mm in females. The glottis at its widest (posterior) point is 6 to 9 mm but can be “stretched” to 12 mm.6 It should be noted that the vocal cords are covered by a thin, adherent mucosa, producing a pearly white appearance. The absence of any submucosa implies that the vocal cords are unlikely to “swell” significantly as there is minimal space to accumulate edema fluid. However, the folds of mucosa and fibrous tissue lying parallel to the true vocal cords just superiorly in the glottis, the vestibular folds or “false vocal cords,” can become edematous. The intrinsic laryngeal musculature functions to open the glottis during inspiration; close the glottis and constrict the superior structures during swallowing; and finely control abduction, adduction, and tension of the true vocal cords during phonation.

Pharyngeal Innervation
Innervation of the pharynx is supplied via sensory and motor branches of the glossopharyngeal nerve (CN IX) and vagus nerve (CN X) (external and internal branches of the superior laryngeal nerves, recurrent laryngeal nerves). The sensory innervation of the nasopharynx is derived from the maxillary division of the trigeminal nerve (CN V), whereas the oropharynx is diffusely innervated by sensory branches from the glossopharyngeal nerve (CN IX). The internal branch of the superior laryngeal nerve pierces the lateral aspect of the thyrohyoid membrane along with the superior laryngeal artery and vein to provide sensation for the base of the tongue, vallecula, epiglottis, aryepiglottic folds, pyriform recesses, and the superior aspect of the true vocal cords. The external branch of the superior laryngeal nerve provides motor to the cricothyroid muscle, a tensor of the true vocal cords. The recurrent laryngeal nerves supply sensation to the vocal cords and tracheobronchial tree as well as motor to all the remaining intrinsic musculature of the larynx. The right recurrent laryngeal nerve passes inferior to the right subclavian artery but the left originates at the level of the aortic arch and loops around the ligamentum arteriosum then both nerves ascend cephalad along the tracheoesophageal groove. This anatomy must be appreciated during esophageal and thyroid surgery and during both cervical and anterior mediastinoscopy, as these structures can be at risk. The larynx receives its blood supply from the superior and inferior laryngeal arterial branches of the superior and inferior thyroid arteries, respectively. These arteries follow the course of the superior and recurrent laryngeal nerves.
The major function of the upper airway is to provide a conduit for the initial inhalation then exhalation of gases to and from the lungs while contributing to multiple other functions (e.g., eating, drinking, speaking, etc). With respect to inhalation, the nasopharynx and posterior pharynx warm and humidify the inspired gas. This aids in maintaining core temperature and protects the more delicate epithelia lining the lower airways from desiccation. The airway epithelium secrets mucus, which coats the airway surface and maintains tissue hydration and also serves to trap particulate matter, bacteria, and viruses. Mucus also contains a number of enzymes with antioxidant, antiprotease, and antibacterial properties.7
Another important role of the airways and their mucus coat is the filtering of inhaled particulate matter by an elaborate defense system that takes advantage of the air-flow characteristics of the upper and lower airways and their associated epithelium. There are three mechanisms at work to produce mechanical filtering of inspired gases.1 The first, inertial impaction, is capable of trapping particulates larger than 10 microns by virtue of the turbulent flow across the mucus lining the passageways. It accomplishes this task in minutes with mucus and saliva eventually swallowed. Gas flow slows within the bifurcating and branching tracheobronchial tree until it becomes more laminar. Particulates impact the airway wall according to particle size (sedimentation). Normally, the particles, including bacteria and similar-sized particles, are trapped in the mucus at this level of more proximal airways and are transported cephalad by the constant motion of the cilia, an apical feature of the respiratory epithelium, at a rate of approximately 2.5 mm per minute in the bronchi but over 5 mm per minute in the trachea. Lower airway mucus is usually cleared in about 24 hours although this can be drastically retarded in disease states such as cystic fibrosis or chronic bronchitis or conditions altering ciliary function or growth, such as tobacco smoking.8 The filtering processes appear effective down to particles approximately 0.01 micron in diameter.
Upper Airway Gas Flow
Gas flow is directly proportional to the pressure gradient (ΔP) and inversely proportional to the resistance. When a gas (or liquid) flows through a straight unbranched tube, flow will usually be laminar and resistance is directly proportional to the viscosity of the gas and inversely proportional to the 4th power of the radius.
Resistance = 8 × length × (viscosity/π) × (radius)4
However, at very high flow rates or when gas flows through an irregular tube or orifice, flow tends to become turbulent and resistance becomes proportional to the density of the gas and inversely proportional to the 5th power of the radius (i.e., changes in airway caliber affect resistance to turbulent flow more than laminar flow). Reynolds number is a dimensionless number that allows estimation of whether a flow is turbulent or laminar.9
Reynolds number = velocity × diameter × density/viscosity
In the airway, Reynolds numbers for air during quiet breathing are <2,000 throughout most of the upper and lower airway (Fig. 24-4). Reynolds numbers >4,000 are associated with turbulent flow and 2,000 to 4,000 is a mixture of laminar and turbulent flows. Helium is a gas with a low density compared to air or oxygen; however, the viscosity of the three is almost equal. A mixture of 80% helium/20% oxygen has a density approximately 0.33 that of air and 0.30 that of oxygen. In conditions of increased turbulent flow in large airways due to a mass or edema, breathing a mixture of helium and oxygen will decrease dyspnea for some patients (this is also why breathing helium changes phonation so that a person may imitate Donald Duck). This applies only to turbulent flows in large airways. Helium does not relieve dyspnea due to increased distal laminar airflow resistance such as in asthma or chronic obstructive pulmonary disease (COPD).

Tracheal and Bronchial Structure
The trachea originates at the cricoid cartilage (at the level of vertebra C6) and extends approximately 10 to 12 cm (females) and 12 to 14 cm (males) to terminate in a bifurcation (carina) at the T4/5 vertebral level (2nd intercostal space, the angle of Louis) (Fig. 24-5). The trachea is 22 ± 1.5 mm (males) to 19 ± 1.5 mm (females) in diameter and consists of 16 to 20 U-shaped cartilaginous rings that are closed posteriorly by fibrous tissue and a longitudinal smooth muscle band, the trachealis muscle.

The right main bronchus is wider (16 vs. 13 mm), shorter (1.5 to 2.5 vs. 4.5 to 5.0 cm) and more vertical than the left. The right main bronchus gives off the upper lobar bronchus then continues as the bronchus intermedius giving off the right middle lobar bronchus and right lower lobar bronchus at the hilum of the lung at T5 (Fig. 24-6). The left main bronchus passes inferiorly and laterally below the aortic arch, anterior to the esophagus and descending thoracic aorta to reach the hilum of the left lung at T6. These dimensions can be quite variable among individuals and chest pathology can drastically change the anatomy.

The lobar bronchi (right upper, right middle, right lower and the left upper, left lower) extend into their segmental bronchi that can be readily visualized during flexible bronchoscopy. The right upper lobe bronchus gives off 3 segmental bronchi (apical, anterior, posterior) (Fig. 24-7), the right middle lobe bronchus splits into 2 segmental bronchi (lateral, medial), and the right lower lobe bronchus divides into a superior segment (directed posteriorly) and a basilar segmental bronchus which divides into 4 segments (medial basal, anterior basal, lateral basal, posterior basal) for a total of 10 segmental branches on the right. The left upper lobar bronchus splits into the superior division with “3” segments (a “fused” apical-posterior and an anterior) and the inferior division or lingual with 2 segments (superior and inferior). The left lower lobe bronchus branches into 4 lower segmental branches (superior, a “fused” anteromedial basal, lateral basal, and posterior basal) for a total of “10” segments on the left. An online interactive bronchoscopy simulator is available to demonstrate this anatomy (Fig. 24-8).


Respiratory Airways and Alveoli
The airways continue to divide into smaller diameter conduits until one arrives at the bronchioles with diameters less than 0.8 mm. At this level, the airways lose all remnants of cartilage and begin the transformation from purely conducting airways to those described as respiratory bronchioles. Respiratory bronchioles eventually divide into the final four generations of alveolar ducts, which then consist primarily of openings into the terminal alveolar sacs. In the descriptive model of Weibel10 (Fig. 24-9A), the trachea branches into 23 generations of airways. The first 15 generations serve as conducting airways and the subsequent 8 generations become sufficiently thin-walled to allow some degree of gas exchange and are called acinar airways. One clinical aspect of this geometric progression of increasingly narrower airways (and blood vessels) by divergence and multiplication is that the overall cross-sectional area and therefore resistance to gas flow (or blood flow) becomes markedly less compared to the resistance of the proximal airway (or blood vessel) (Fig. 24-9B). This has an important impact on distribution of gas and blood flow, flow velocity, and, hence, transit time through key areas of gas exchange.

The interior of the trachea is lined with ciliated columnar epithelium, goblet cells (responsible for mucus production) (Fig 24-10), and with interspersed specialized chemical and tactile neuroreceptors. The lining of the airways transitions from pseudostratified columnar epithelia in the larger bronchi to a thinner cuboidal ciliated variety in the small bronchi. The airway epithelium and submucosa also contain lymphocytes, mast cells, and a variety of neuroendocrine cell types. The next layer consists of circumferential bands of smooth muscle cells and a connective tissue layer containing submucosal glands and plates of cartilage (replacing the solid cartilage rings in the very large airways) (Fig. 24-11). The outermost layer is a loose adventitial shell with lymphatic vessels, sympathetic and parasympathetic nerves, and nourishing blood vessels.


The respiratory bronchioles terminate in a pulmonary acinus, which has the appearance of a cluster of grapes on a network of stems. Each acinus may contain multiple alveolar ducts communicating with 2,000 alveoli arranged in a ring-like, honeycomb network. The alveolus is considered the primary site of gas exchange between the blood and gas in the lung. The alveolar septa are about 5 to 8 microns thick and are opposed by an alveolar surface on either side with the alveolar capillary bed sandwiched inside. The walls of the alveoli are extremely thin, between 0.1 and 0.2 microns, a feature that promotes rapid equilibration of gas by diffusion with the pulmonary capillary blood. In addition, gas can exchange between alveoli through pores of Kohn. There are approximately 300 million alveoli in the human lung, which provides an extraordinary surface area for gas exchange (e.g., 70 m2).
There are three major cell types found in the alveolus: alveolar type I cells, alveolar type II cells, and alveolar macrophages. However, there are other cell types found under certain conditions in the lung (e.g., inflammation). Alveolar type I cells are squamous epithelial cells that cover most of the alveolar surface. These nucleated cells have few cytoplasmic organelles and a sparse cytoplasm splayed out in sheets over the alveolar surface forming a thin barrier between the air space and the pulmonary capillary endothelium. Alveolar type II cells are fewer in number, somewhat spherical, and coated on their apical surface with microvilli. In contrast to type I cells, alveolar type II cells possess many organelles including multilayered granular structures called lamellar bodies (Fig. 24-12). These lamellar bodies are the source of pulmonary surfactant, a lipoprotein coating the interior surface of the alveolus and capable of significantly reducing the surface tension of the alveolus air-surface interface. Surface tension reduction is considered an important physical mechanism to reduce any tendency for alveolar collapse at very low lung volumes.

The immune defenses of the lung are extremely important because of the direct exposure of this organ to the external environment via the airways. There are a number of excellent reviews of the immune function of the lung but it is important to realize that there are many questions unanswered about how the lung responds to invasion and inflammation. Yet from a clinical standpoint, the pulmonary inflammatory response will greatly influence the perioperative management of the surgical patient. A few major defensive cell types residing in the alveolar spaces and interstitium are worth mentioning. Alveolar macrophages are derived from bone marrow monoblast precursor cells and migrate to the lung parenchyma (Fig. 24-13).11 Alveolar macrophages are free to move over the surface of the alveolus and phagocytize foreign material that enters the alveolus including bacteria and particulates. Macrophages are cleared either through the lymphatics or are carried up and expelled via the airways. Lymphocytes, largely T lymphocytes, are widely distributed in the normal lung within paratracheal and hilar lymph nodes, in the interstitium of the bronchial tree as nodules or individual cells and in the alveolar walls. They play a critical role in the lung’s primary immune response to inhaled antigens. Under some pathologic conditions in the lung, it is becoming apparent that an exaggerated inflammatory response and the activity of these cells and others may be harmful to the lung; the acute respiratory distress syndrome (ARDS) and emphysema are examples.

Pulmonary Circulation
Although the blood flow through the pulmonary circulation is normally equal to the blood flow though the systemic circulation (a major exception being intracardiac shunting when it exceeds systemic circulation), the pressures in the pulmonary circulation are normally lower than the systemic circulation because the pulmonary vascular resistance (PVR) is lower than the systemic resistance (approximately one-sixth of systemic resistance). This is because the pulmonary vessel walls contain less elastic and muscular tissue than systemic vessels of corresponding caliber. Pulmonary arterioles contract rapidly in response to hypoxemia in the alveolus and to a lesser extent to hypoxemia in mixed venous blood. This hypoxic pulmonary vasoconstriction (HPV) is unique to the pulmonary circulation (systemic arterioles dilate in response to hypoxia) and permits regional matching of perfusion to ventilation.
Like all endothelium, the vascular luminal wall of the pulmonary capillaries is lined by glycocalyx, a microcilial layer that acts as a molecular sieve.12 This 0.1-micron layer prevents adhesion of platelets and leukocytes and is thought to trap larger molecules close to the endothelial membrane and locally increase the oncotic pressure. The glycocalyx covers the pores between endothelial cells and acts as a molecular sieve to control fluid flux. A healthy glycocalyx is important to prevent edema formation in the lung. However, the glycocalyx is damaged by inflammation and ischemia-reperfusion and this may contribute to the increased flux of fluid into the pulmonary extracellular matrix in these conditions.
The air passages receive their blood supply from systemic bronchial arteries down to the level of the respiratory bronchioles. Only one-third of the bronchial circulation returns to the systemic venous system, the remainder drains into the pulmonary veins and this constitutes the largest portion of the normal extrapulmonary venoarterial shunt. This bronchial shunt is less than 1% of the cardiac output in healthy individuals but may increase to 10% in bronchiectasis, emphysema, and some congenital cardiac conditions.
Thorax and Muscles of Respiration
The lungs are contained within the thorax. The bony thorax is composed of the 12 ribs, the sternum anteriorly and the thoracic vertebral column posteriorly. The caudal end of the thorax is formed by the diaphragm and the cranial end of the thorax is the thoracic inlet, within the ring formed by the first ribs, containing the trachea, esophagus, and the neurovascular supply to the head and arms. Bulk movement of air into and out of the lungs occurs as a result of changes in intrathoracic pressure created by rhythmic changes in the volume of the thorax. Expansion of the chest cavity occurs when three respiratory muscle groups work in concert. The diaphragm, intercostal muscles, and the accessory muscles (sternocleidomastoids, scalenes) are controlled by the respiratory centers of the brain to contract in a rhythmic pattern designed to match ventilation to gas exchange requirements. The abdominal musculature (rectus abdominis, external oblique, internal oblique, and transversus abdominis) can be recruited when more force is required for exhalation, although abdominal muscle tone may stabilize the rib cage during inspiration as well.1
Inspiration
The diaphragm is unique in that its muscle fibers radiate from a central tendinous structure to insert peripherally on the ventrolateral aspect of the first three lumbar vertebrae, the aponeurotic arcuate ligaments, the xiphoid process and the upper margins of the lower six ribs. Its motor innervation is solely from the right and left phrenic nerves, which originate from the 3rd, 4th, and 5th cervical spinal nerves. In the relaxed state, it forms a pronounced “dome” that closely apposes the chest wall for some distance before arching across. Contraction of the diaphragm causes a large caudal displacement of the central tendon resulting in a longitudinal expansion of the chest cavity. Simultaneously, its insertions on the costal margins cause the lower ribs to rise and the chest to widen. This diaphragmatic motion is responsible for the majority of quiet respiration. As the dome descends, it displaces the abdominal contents caudally. The fall in pleural pressure and accompanying lung expansion produce an increase in abdominal pressure and outward movement of the abdominal wall. The supine and Trendelenburg positions or surgical retractors can significantly interfere with this abdominal motion especially in the morbidly obese, necessitating controlled ventilation under anesthesia.
The intercostal muscles are thin sheet-like muscles with origins and insertions between the ribs. The internal intercostal muscles have their fibers oriented obliquely, caudally, and dorsally, from the rib above to the rib below. The external intercostal muscles have their fibers oriented obliquely, caudally, and ventrally, from the rib above to the rib below. All intercostals are innervated by the intercostal nerves running in the neurovascular bundle under the inferior lip of each rib. The contraction of the external intercostal muscles produces an inspiratory action by elevating the upper ribs to increase the anteroposterior dimensions of the chest in a “bucket-handle” motion. The lower ribs are also elevated by virtue of the force applied and their point of rotation to increase the transverse diameter of the thorax. The internal intercostals apply their force in such a direction as to rotate the ribs downward, decreasing the thoracic anteroposterior dimension to aid in active expiration (when required) and cough. In general, the intercostal muscles do not play a major role in quiet respiration but do in exercise or other conditions requiring high levels of ventilation.
The principal accessory respiratory muscles are the sternocleidomastoid and scalene muscles. The scalene muscles originate from the transverse processes of the 4th through the 8th cervical vertebrae and slope caudally to insert on the first two ribs. Their contraction during periods of high ventilatory demand, elevates and fixes the cephalad rib cage during inspiration. Similarly, the sternocleidomastoid muscles elevate the sternum and increase the longitudinal dimensions of the thorax.
Expiration
Expiration is a passive process in quiet breathing and is largely the response to relaxation of the inspiratory muscles and the balance of forces generated by the elastic recoil of the lungs and chest wall. When high levels of ventilation are required as in exercise or if airway resistance increases (as in exacerbations of asthma or COPD), the expiratory phase becomes an active process with forceful contraction of the rectus abdominis, the transverse abdominis, and the internal and external oblique muscles. The contraction of the abdominal musculature retracts the abdominal wall and pulls the lower ribs downward, which increases intraabdominal pressure and accelerates the cephalad displacement of the diaphragm during exhalation. The internal intercostal muscles depress the rib cage and provide a minor contribution to forced expiration. Innervation of the abdominal musculature is from thoracic nerves 7 through 12 and the 1st lumbar nerve.
Like most skeletal muscles, the diaphragm and intercostal muscles contain a heterogeneous mix of fiber types. The diaphragm has between 49% and 55% type I (slow-oxidative) fibers, the reminder a mix of the “faster high activity” types IIA and IIB fibers.13 The types of skeletal muscle fibers are distributed fairly evenly throughout the diaphragm. Of note, the respiratory muscles retain the ability to adapt to stress and training. This includes responses to lung pathology which might seem maladaptive. Emphysema is a good example. The diaphragm undergoes changes at the sarcomere level, physically “losing” contractile units as hyperinflation of the lungs leads to increasing thoracic dimensions and “flattening” of the diaphragm.14 Loss of sarcomeres in series with the central tendon may help to restore the mechanical advantage of the optimal length-tension relationship for the muscle.
Respiratory Mechanical Function
The basics of mechanical function of the respiratory system are the interaction of two opposing springs: the chest wall, which at rest is trying to expand, and the lungs, which at rest are trying to contract. (Fig. 24-14) The lungs and chest wall move together as a unit. This is made possible by the enclosed, air-tight thoracic cavity where the outer surface of the lungs and its visceral pleura are in close proximity to the parietal pleura covering the inner surface of the chest wall and the mediastinal structures. Changes in the intrathoracic volume are only possible because the inside of the lung is in continuity with the ambient atmosphere outside the thorax via the trachea and pharynx. The intimate contact between the layers of pleura is maintained by a negative intrapleural pressure generated in part by the intermolecular forces of the pleural fluid excluding gas from this space. This lubricating fluid allows freedom of the pleural layers to slide over one another but highly resists separation of the layers much like two panes of glass with a thin layer of water between them.

Normally, the intrapleural pressure is about −5 cm H2O when the respiratory system is at equilibrium. Because of the deforming effect of gravity on the lung parenchyma, there is a vertical gradient of intrapleural pressure. This gradient is largest in the seated or upright position (Fig. 24-15). The volume of gas contained in the lungs at this resting point is termed functional residual capacity (FRC). For a healthy young adult male, total lung capacity (TLC) will be approximately 6 to 6.5 L and FRC will be 2.5 to 3 L. The oxygen contained in the FRC (500 to 600 mL) is the only reservoir of oxygen in the body.

Pathologic conditions such as the introduction of air or blood into the intrapleural space can rapidly disrupt this lung–chest wall interaction, leading to a compromise in respiratory function but also interfere with cardiovascular function. Examples of disruption of the intrapleural space would be a pneumothorax, empyema, pleural effusion, or bronchopleural fistula.
Lung Volumes and Spirometry
By convention, the static and dynamic subdivisions of gas contained within the lung are given a common nomenclature of volumes and capacities (Table 24-1 and Fig. 24-16). Volumes are most commonly measured by spirometry (Figs. 24-17 and 24-18) and capacities are then calculated as the sum of specific volumes. Simple spirometry can give all of the volumes and capacities listed in Table 24-1 except FRC, TLC, and residual volume (RV), all of which require a separate measurement of RV. RV can be measured by wash-in or washout dilution calculations using a relatively insoluble gas such as nitrogen or helium and a closed breathing circuit. In modern pulmonary function labs, these laborious techniques have largely been replaced by whole-body plethysmography, which is both simpler and more accurate (Fig 24-19), which measures FRC and this can be used to calculate RV by subtraction of expiratory reserve volume (ERV) measured by spirometry.





Spirometry measurements are commonly reported as “observed” (or measured) and “predicted” (Fig. 24-20). Predictions are based on population statistical means, which take into account age, sex, and height. For example, an observed forced expiratory volume in 1 second (FEV1) of 1.0 L for an 85-year-old male, 152 cm in height (5-ft tall) is 85% predicted (within the normal range of 100% ± 20%) but and FEV1 of 1.0 L for a 30-year-old male, 182 cm (6 ft) is 20% predicted, which would be consistent with severe end-stage lung disease. The FEV1, forced vital capacity (FVC), and their ratio (FEV1/FVC) are the most useful spirometry measurements for the anesthesiologist to assess the severity of a patient’s lung disease or to evaluate a patient’s operability for lung resection surgery.15

In addition, complete pulmonary function testing in the laboratory will commonly report measurements of volume ratios, flows, lung resistance, lung diffusion capacity for carbon monoxide (DLCO) and other measurements. The majority of these other values, although useful for distinguishing among different types and severities of pulmonary diseases in clinical chest medicine, are not commonly used in anesthesia. The exception is the DLCO which is a measurement of lung parenchymal function (i.e. alveolo-capillary gas transfer).
Closing Capacity and Closing Volume:
The key to understanding the complex changes which develop in the respiratory system during anesthesia is to appreciate the relationship between FRC and closing capacity (CC). CC is the sum of closing volume (CV) and RV. CV is the lung volume below which small airways begin to close (or at least cease to contribute expiratory gas) during expiration. Closure of small airways in the basal portions of the lung during deep expiration is a normal phenomenon due to the gravity-dependent increase in pleural pressure at the bases and due to the lack of parenchymal support in distal airways. CV and CC are not commonly measured in the pulmonary function lab. Measurement is either by a wash-in technique with a small bolus of an insoluble tracer gas such as 133Xe slowly inhaled then exhaled from RV (Fig. 24-21) or by nitrogen washout after inspiration of a breath of oxygen from RV. Normal values for CC in seated healthy young adults are 15% to 20% of vital capacity (VC).5 CC increases with age due to loss of structural parenchymal support tissue in the lung and an increase in RV. FRC increases slightly with age but the increase is greater for CC (Fig. 24-22).16 CC changes very slowly over time. However, FRC changes on a minute-to-minute basis as the mechanical advantage of the two springs (lung and chest wall) which determine it change. CC exceeds FRC in the supine position at age 45 and in the upright position by age 65. During anesthesia, a decrease in the elastic recoil of the chest wall due to the muscle-relaxing effects of almost all general anesthetics (with the possible exception of ketamine) and neuromuscular blockers causes FRC to decrease and it will often fall below CC.17 Similarly, an increase in elastic recoil of the lung due to fluid retention in the pulmonary parenchyma will lower the FRC. When an alveolar unit falls below its CC, even for a brief period during one respiratory cycle, the concentration of oxygen (PAO2) in that unit falls slightly. This results in the increase of venoarterial admixture (“shunt”; see the following text) and decrease in arterial oxygen tension (PaO2) seen in the elderly and during general anesthesia. When a region of the lung is kept below its CC, the loss in volume will eventually lead to atelectasis (Fig. 24-23) as the gas trapped in the alveoli is absorbed. A large part of the anesthesiologist’s job in the perioperative period is restoring the balance between FRC and CC. Because CC cannot be changed, this involves improving FRC by a variety of techniques to improve the mechanical advantage of the chest wall. These techniques may include ensuring adequate reversal of neuromuscular blockers, upright positioning, regional analgesia and possibly the use of positive end-expiratory pressure (PEEP) or continuous positive airway pressure (CPAP). The physiologic differences between PEEP and CPAP are subtle. However, by common usage, when positive pressure is applied during expiration to the airway of a patient who is having positive pressure ventilation, this applied airway pressure is referred to as PEEP. When a patient is breathing spontaneously, an applied airway pressure is referred to as CPAP.



Compliance
Compliance is the change in lung volume for a given change in airway pressure. It is the reciprocal of “elastance.” Monitoring changes in respiratory compliance is extremely important in ventilated patients as an early warning of changes in the lung or chest–abdominal wall complex that may negatively affect gas exchange. Compliance of the respiratory system (CRS) is measured as the change in lung volume (ΔV) divided by the change in airway pressure (ΔP), this represents the difference between alveolar pressure, at a given lung volume, and ambient (atmospheric) pressure.
CRS = ΔV/ΔP
The compliance of the respiratory system is dependent on the interaction of the compliance of the lung itself (CL) and the compliance of the chest wall (Ccw). These two springs act similar to series capacitors in an electrical system, that is, storing energy, and the reciprocal of respiratory compliance is the sum of the reciprocals of CL and Ccw:
1/CRS = 1/CL + 1/Ccw
CL is calculated as ΔV/ΔP, where ΔP = alveolar pressure − intrapleural (“transmural”) pressure
Ccw is calculated as ΔV/ΔP, where ΔP = intrapleural pressure − ambient pressure
Intrapleural pressure is not easy to measure directly in the clinical setting. Esophageal pressure, from a balloon manometer, is commonly used as an approximation of intrapleural pressure in respiratory research. As the respiratory system inflates, both the lung and chest will produce their own unique compliance curves (Fig. 24-24).

Factors affecting CL include the following:
1. Lung volume. Compliance is greatest at FRC and remains at this level until the lung inflates or deflates to approximately 15% of TLC above or below FRC, that is, the slope of CRS is the steepest in this range, which includes the range of normal tidal volume breathing. Compliance is related to the normal FRC for a given lung and can be corrected for normal lung volume as the “specific compliance” (compliance/FRC), which is relatively constant at all ages.
2. Surface tension of the alveoli. This is probably the major factor determining lung recoil and CL. A lung that is filled with water is actually more compliant than a normal lung because the air-fluid surface tension interaction is lost. Similarly, a lung that is depleted of surfactant is less compliant than normal. Without surfactant, the alveoli would be expected to behave like communicating bubbles and conform to the Laplace equation:
P = 2T/R
Where P is the gas pressure within a bubble, T is the surface tension of the wall, and R is the radius of the bubble. If the radius is decreased without a change in surface tension, the pressure will increase in the bubble and a small bubble will empty into a larger bubble (in the lung, this would lead to atelectasis). However, in the lung, surface tension decreases as the radius of the alveoli decreases and this opposes the collapse of smaller lung units. The exact mechanisms by which surfactant causes this effect are debated.18 It could be related to a tighter packing of surfactant molecules as the radius decreases or the formation of surfactant multilayers. Other factors which affect the CL include pulmonary blood volume and interstitial edema.
Factors affecting Ccw include posture, obesity, ossification of the costal cartilages, and scarring of the skin. The interaction of the compliances of these two springs produces the characteristic sigmoid pattern of the compliance curve of the respiratory system (see Fig. 24-24, solid line). At FRC, which is normally the relaxation volume of the respiratory system, the pull of the two springs in opposite directions balance one another. In a normal healthy individual, as the respiratory system inflates to 60% of TLC, the chest wall is aiding the muscles of respiration to inflate the lungs. However, as the lung inflates above this volume, the muscles of inspiration must work to distend both the lungs and the chest wall.
Dynamic compliance is the ΔV/ΔP of the respiratory system measured at the instant gas flow. In a ventilated patient, this ΔP will = peak airway pressure − PEEP. This reflects the normal behavior of the respiratory system but will include the effects of airway resistance and the normal hysteresis of the lung parenchyma (hysteresis in this context refers to the tendency of an elastic material to resist change of shape both during stretch and contraction). Dynamic compliance will be affected by both the frequency of respiration and the velocity of gas flow.
Static compliance is the ΔV/ΔP of the respiratory system measured at a point of no gas flow and when the pressure gradient has been allowed to equilibrate in the entire airway. This is difficult, but not impossible, to achieve in an awake subject by relaxing at end-inspiration against a closed airway. However, it is simple to measure in an anesthetized and paralyzed patient during ventilation in a volume-controlled mode (with a fixed inspiratory flow) by using an end-inspiratory pause. The ΔP for static compliance will = plateau airway pressure − PEEP.
Both static and dynamic compliances provide useful information for the anesthesiologist. The static compliance reflects more the actual distending pressure in the patient’s alveoli. The difference between the two reflects the effects of airway resistance. During pressure-control ventilation, with a decreasing inspiratory airflow pattern, there will not be a discernible difference in the peak or plateau in the airway pressure so distinguishing between static and dynamic compliance is clinically difficult (Fig. 24-25). This negative, that is, loss of monitoring, aspect of pressure–control ventilation is largely compensated by the ability of pressure–control ventilation to more uniformly distribute gas flow in patients with COPD who have large differences in regional compliance within the lung (see the following text).

The inflation and deflation limbs of the compliance curve of the respiratory system are different (Fig. 24-26). For dynamic compliance, this is due to both airway resistance and lung hysteresis. These curves combine to produce the familiar “pressure-volume loop” (or “PV” or “ΔV/ΔP” or “compliance” curve) of the lung displayed by many modern anesthetic machines and its shape is determined mainly by the dynamic compliance of the respiratory system. The gap between the expiratory and inspiratory limbs of the combined curve will widen as tidal volume and respiratory rate increase. It is possible to generate a ΔV/ΔP curve using static compliance if the lung is slowly inflated in a stepwise fashion. This curve will also show a gap between inspiration and expiration due mainly to lung hysteresis. This gap will be smaller than that for the dynamic ΔV/ΔP curve.19 An automated breath-by-breath calculation of compliance is displayed by the ventilation monitors of many modern anesthetic machines. It is difficult for these clinical monitors to measure at a true static “no flow” point at end-inspiration and therefore measured compliance changes may be truly due to increased tissue elastance (e.g., atelectasis or pulmonary edema) but may also be affected by changes in dynamic compliance (airway resistance), for example, bronchospasm or secretions.

Resistance
Respiratory system resistance is a complex and important topic. It is important because, in the perioperative period, complications such as bronchospasm or secretions in an endotracheal tube or partial circuit obstruction will present primarily as increased resistance. It is complex because there are many types of resistance that contribute to the overall resistance of the respiratory system. When anesthesiologists think of respiratory resistance, they are mainly thinking of the nonelastic resistance to gas flow and they are primarily thinking of frictional airflow resistance. Nonelastic resistance is a major component of the work of breathing and because it cannot be stored, it is lost and dissipated as heat. Nonelastic resistance also includes the resistance of lung and chest wall tissue to deformation and compression of intrathoracic gas. Elastic resistance is the recoil of the lung and chest wall, and because it is recovered during expiration, it does not contribute to the work of breathing.
Respiratory resistance (RRS) is calculated as the pressure gradient divided by the inspiratory flow. During a constant flow situation such as volume–control ventilation, this would be calculated from Figure 24-25 as:
RRS (cm H2O/L per second) = PPeak − PPlateau (cm H2O)/inspiratory gas flow (L per second)
However, normal spontaneous ventilation and pressure–control ventilation have variable flow rates and a mean gas-flow rate approximation is necessary to calculate respiratory resistance. Airflow resistance in normal healthy individuals breathing spontaneously is approximately 1 cm H2O/L per second. This can increase to 5 to 10 cm H2O/L per second in COPD and asthma.20 Breathing through an 8-mm internal diameter endotracheal tube at a flow of 1 L per second creates a resistance of approximately 5 cm H2O/L per second and this increases to 8 cm H2O/L per second for a 7-mm endotracheal tube.21
To quantitatively measure respiratory resistance in the operating room is not simple because it is difficult to separate respiratory system resistance from apparatus resistance. In the pulmonary function laboratory, this is done with plethysmography and a variety of flow-interrupter techniques. Fortunately, it is relatively simple to monitor changes in respiratory resistance in the operating room by following changes in dynamic compliance.
Gas flow during respiration is a mixture of turbulent and laminar flows and the turbulent/laminar interface moves in the air column during the respiratory cycle. Laminar flow occurs beyond the 11th generation airways. Turbulent flow in the larger conducting airways aids clearing of secretions by coughing. In a healthy person, frictional airflow resistance is mainly due to larger airways: mouth and pharynx 40%, larynx and large airways 40%, small airways (<3-mm diameter) contribute 20%.22 However, changes in airflow resistance are most commonly due to changes in the caliber of the small airways. Small airway caliber can be decreased by contraction of smooth muscle in the airway wall or by compression (due to reversal of the normal transluminal pressure gradient in the collapsible distal airways).
Airway resistance is inversely proportional to lung volume and increases exponentially as the lung deflates below FRC. The application of PEEP or CPAP to patients who have a decreased FRC will benefit their respiration not only by raising their FRC above CC but also by decreasing their respiratory resistance, and thus their work of breathing, at a higher lung volume.
An increase in resistance to inspiration is detected by the muscle spindles in the diaphragm and leads to an increased force of contraction. This spinal reflex is preserved during anesthesia. In the awake patient, this reflex is augmented by a conscious cortical response that also increases the force of inspiration.23 Increased expiratory resistance does not normally initiate a response if the resistance is <10 cm H2O. The FRC is increased passively by the increased resistance until the increased elastic recoil balances the increased work of expiration. However, the ensuing increased intrathoracic pressure may decrease venous return and cardiac output. Patients tolerate increased airway resistance by increasing the work of breathing and in the short term will usually maintain a normal arterial CO2 (PaCO2). Eventually, a major increase in airway resistance may lead to respiratory muscle fatigue and the PaCO2 will start to rise. An elevated PaCO2 in a patient with increased respiratory resistance who has a normal baseline PaCO2 is an ominous sign of impending respiratory failure.
The Equal Pressure Point
Expiratory respiratory resistance will normally be lower than inspiratory resistance because the lung is at a larger volume at all stages of expiration than inspiration (see Fig. 24-26). However, there are situations when expiratory resistance exceeds inspiratory resistance, these include the following: during a forced expiration (or cough) in a normal patient, during quiet breathing in some patients with severe emphysema (see the following text), and during forced expiration in some patients with an intrathoracic tracheal tumor or tracheobronchial compression from a mediastinal mass. In these instances, where expiratory resistance exceeds inspiratory resistance, the underlying cause is dynamic airway compression, which creates a moving flow-limiting narrowing in the airway called the equal pressure point (EPP). In Figure 24-27 at FRC before inspiration, (a) airway pressure throughout is zero (no-flow situation) because the intrapleural (transpulmonary) pressure is −5 cm H2O there is a net +5 cm H2O pressure distending the airways and alveoli. As inspiration begins, (b) intrapleural and alveolar pressure falls by 3 cm H2O and flow begins. Because of the pressure drop along the airway, the pressure will be negative in the airway but less than the alveolar pressure. This increases the distending pressure of the airways in this case from +5 to +6 cm H2O. At end-inspiration, (c) the distending pressure in the no-flow condition is +8 cm H2O and the pressure through the airway returns to zero. During quiet expiration, the recoil intrapleural pressure returns to −5 cm H2O and this creates a net pressure in the alveolus of +3 cm H2O, which diminishes proximally as air flows out the tracheobronchial tree. The downstream distending pressure falls along the airway (represented by +6 cm H2O in Fig. 24-27D). Because this is a dynamic process, due to tissue resistance, the airway caliber will normally be larger at a given point for the same distending pressure during expiration than during inspiration. In a no-flow situation, the same distending pressure will result in equivalent airway diameters. During a forced expiration, (e) the airway pressures increase [in this case by 24 cm H2O, resulting in a net intrapleural pressure of +16 cm H2O for same lung volume as (c)] and a gradient is created along the expiratory air column. At the point where the intrapleural pressure equals the air column distending pressure (+16 cm H2O), an EPP is created. The airway will narrow proximal to this point (+15) to the thoracic outlet (+14). This becomes the flow-limiting point of expiration and no amount of increased effort can increase the expiratory flow at a particular lung volume because the driving pressure is fixed by the difference between the alveolar and intrapleural pressures [8 cm H2O in (e)]. This EPP allows the creation of a point of gas-flow acceleration and turbulence in the expiratory air column during normal coughing that has a Bernoulli effect (decreased lateral pressure in a region of increased flow velocity) to detach secretions from the tracheobronchial walls. During forced expiration, as lung volumes decrease and airway pressures decrease, intrapleural pressures are maintained and the EPP will move distally in the airway.24

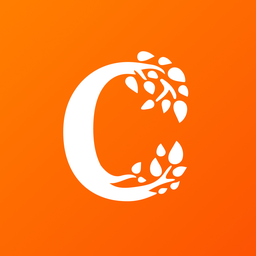
Full access? Get Clinical Tree
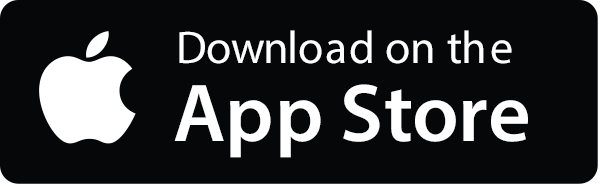
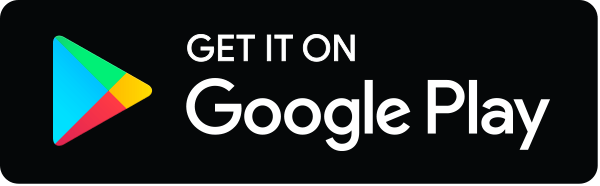