Acknowledgment
The authors wish to thank Dr. Michael M. Todd for the inspiration for Figs. 9.4, 9.5 , and 9.6 and Dr. Mark Zornow for permission to use Figs. 9.7 A and B .
The intraoperative fluid management of neurosurgical patients presents special challenges for the anesthesiologist. Neurosurgical patients often experience rapid changes in intravascular volume caused by hemorrhage, the administration of potent diuretics, or the onset of diabetes insipidus. The administration of volatile anesthetics and potent vasodilators during surgery may contribute to decreased cardiac filling pressures without causing actual changes in intravascular volume. In the midst of this dynamic situation, the anesthesiologist often faces the additional concern of minimizing increases in cerebral water content and, thus, intracranial pressure. Intracranial hypertension secondary to cerebral edema is now known to be one of the most common causes of morbidity and mortality in the intraoperative and postoperative periods.
In this chapter, we examine some of the physical determinants of water movement between the intravascular space and the central nervous system. Then we address specific clinical situations and make suggestions for the types and volumes of fluids to be administered.
Osmolality, oncotic pressure, and intravascular volume
Osmolality
Osmolality is one of the four colligative properties of a solution. (The other three are vapor pressure, freezing point depression, and boiling point elevation.) The addition of 1 osmole of any solute to 1 kg of water causes the vapor pressure to fall by 0.3 mmHg, the freezing point to drop by 1.85° C, and the boiling point to rise by 0.52° C. The colligative properties are determined solely by the number of particles in solution and are independent of the chemical structure of the solute. The solute may exist in either an ionized or a nonionized state, and the size (molecular weight) of the solute is of no importance. Although it may seem counterintuitive, equimolar concentrations of glucose, urea, and mannitol have the same effect on the colligative properties of a solution. Osmolality is strictly a function of the number of particles in solution.
For physiologic solutions, osmolality is commonly expressed as milliosmoles (mOsm) per kilogram of solvent , whereas the units of measure for osmolarity are milliosmoles per liter of solution . For dilute solutions (including most of those of physiologic importance), the two terms may be used interchangeably. Osmolarity can be calculated if the molecular weight of the solute and its tendency to disassociate in solution are known ( Boxes 9.1 and 9.2 ). The osmolarities of some commonly used intravenous fluids are listed in Table 9.1 .
Fact: The molecular weight of NaCl is 58.43 g/mol.
Fact: A 0.9% solution of NaCl contains 9 g of NaCl per 1000 mL of solution.
The first step is to calculate the molarity of the 0.9% solution. To do this, we divide 9 g/L by 58.43 g/mol, which equals 0.154 mol/L or a 154 mmol/L solution of NaCl. Because each molecule of NaCl disassociates in water into a Na + and a Cl − ion, we multiply the molar value by two to get an osmolarity of 308 mOsm/L. This value corresponds to the osmolarity listed on any container of 0.9% saline.
Calculate the osmotic pressure generated by a 1 mOsm difference in osmolarity at body temperature and express the results in millimeters of mercury.
Formula for calculation of osmotic pressure is as follows:
where:
C = 0.001 mol/L (ie, 1 mOsm/L)
R = 0.08206
T = 273° K + 36° K = 309° K (body temperature)
Therefore:
Fluid | Osmolarity (mOsm/L) | Oncotic Pressure (mmHg) |
---|---|---|
Lactated Ringer’s solution | > 273 | 0 |
D 5 lactated Ringer’s solution | 525 | 0 |
0.9% saline | 308 | 0 |
D 5 0.45% saline | 406 | 0 |
0.45% saline | 154 | 0 |
20% mannitol | 1098 | 0 |
Hetastarch (6%) | 310 | 31 |
Dextran 40 (10%) | ≈ 300 | 169 |
Dextran 70 (6%) | ≈ 300 | 69 |
Albumin (5%) | 290 | 19 |
Plasma | 295 | 26 |
Osmolarity is important in determining fluid movement between various physiologic compartments because of the osmotic pressure that is generated when solutions of unequal osmolarity are separated by a membrane permeable to water but not to solutes. According to the second law of thermodynamics, which states that all systems spontaneously change to maximize entropy, water has a tendency to move from the solution of lower osmolality, across the membrane, and into the solution of higher osmolality ( Fig. 9.1 ).
This process continues until the solutions are of equal osmolality or the hydrostatic pressure is sufficient to preclude any further net flow of water across the membrane. The hydrostatic pressure that can be generated by osmolar differences is formidable and may be calculated by the following equation:
where π is osmotic pressure in atmospheres, C is concentration of all osmotically active solutes in the solution (in moles per liter), R is gas constant (0.08206 liter-atm/mole-degree), and T is temperature in degrees Kelvin (° K).
On the basis of this formula, a pressure of more than 19 mmHg is generated for each milliosmole difference across a semipermeable membrane (see Box 9.2 ). Thus, osmolar differences can provide a potent driving force for the movement of water between the intracellular and extracellular spaces and, as seen later, across the blood–brain barrier. Although osmolar gradients can be produced by administering hypo-osmolar or hyperosmolar fluids, these gradients are fleeting; and water moves from one compartment to another so that all body fluids are again of equal osmolarity.
Oncotic Pressure
Oncotic pressure is the osmotic pressure generated by solutes larger than an arbitrary limit (usually 30,000 molecular weight [MW]). Albumin (69,000 approximate MW), hetastarch (480,000 mean MW), dextran 40 (40,000 mean MW), and dextran 70 (70,000 mean MW) are compounds of clinical interest that are capable of exerting oncotic pressure. Reported values for the oncotic pressures of plasma, mannitol, albumin, and hetastarch are listed in Table 9.1 . , The oncotic pressure produced by all plasma proteins (eg, albumin, globulins, fibrinogen) accounts for less than 0.5% of total plasma osmotic pressure. The oncotic pressure of various solutions can be easily measured with an electronic pressure transducer and a membrane that is freely permeable to low-molecular-weight (LMW) solutes but that prevents the passage of particles greater than 30,000 MW ( Fig. 9.2 ).
Determinants of Fluid Movement between Vasculature and Tissues
Nearly 100 years ago, Ernest Starling described the forces that determine the movement of water between tissues and the intravascular space. This description was subsequently formalized in what is now known as the Starling equation, as follows:
where Q f is the net amount of fluid that moves between the capillary lumen and the surrounding extracellular space (interstitium), K f is the filtration coefficient for the membrane, S is the surface area of the capillary membrane, P c is the hydrostatic pressure in the capillary lumen, P t is the hydrostatic pressure (usually negative) in the extracellular space of the surrounding tissue, σ is the coefficient of reflection—this number, which can range from 1 (no movement of the solute across the membrane) to 0 (free diffusion of the solute across the membrane), quantitates the “leakiness” of the capillary and is different for vessels in the brain and those in peripheral tissues, π c is the oncotic pressure of the plasma, and π t is the oncotic pressure of the fluid in the extracellular space.
Capillary pressure, tissue pressure (negative in nonedematous tissues), and tissue oncotic pressure all act to draw fluid from the capillaries and into the extracellular space of the tissue ( Fig. 9.3 ). In peripheral tissues, the only factor that serves to maintain intravascular volume is the plasma oncotic pressure, which is produced predominantly by albumin and to a lesser extent by immunoglobulins, fibrinogen, and other high-molecular-weight (HMW) plasma proteins (see Fig. 9.3 ).
Under most circumstances, the sum of the forces results in a Q f value that is slightly greater than zero, indicating a net outward flux of fluid from the vessels into the tissue extracellular space. This fluid is cleared from the tissue by the lymphatic system, thereby preventing the development of edema ( Fig. 9.4 ).
The clinical effects of altering one or more of the variables in the Starling equation may frequently be observed in the operating room. Many patients who have been resuscitated from hemorrhagic hypovolemia with large volumes of crystalloid solutions demonstrate pitting edema, caused by a dilution of plasma proteins. This results in a decrease in intravascular oncotic pressure (π c ). In the presence of relatively unchanged capillary hydrostatic pressure, an increased movement of fluid from the vasculature into the tissues occurs. When this fluid flux exceeds the drainage capacity of the lymphatics, clinically apparent edema results.
Another example of the Starling equation in action is the facial edema that is often seen in patients who have been placed in the Trendelenburg position for prolonged periods. In this case, the edema is due not to a decrease in plasma oncotic pressure but rather to an increase in the capillary hydrostatic pressure (P c ), favoring an increased transudation of fluid into the tissue.
Fluid Movement between Capillaries and the Brain
The Starling equation describes the factors that govern fluid movement between the intravascular and peripheral extracellular spaces (eg, the interstitium of lung, bowel, and muscle). However, the brain and spinal cord are unlike most other tissues in the body in that they are isolated from the intravascular compartment by the blood–brain barrier. Morphologically, this barrier is now thought to be composed of endothelial cells that form tight junctions in the capillaries supplying the brain and spinal cord. Endothelial cells are surrounded by the layer of pericytes delineated by the foot processes of glial cells. In the normal brain, these tight junctions severely limit the diffusion of molecules between the intravascular space and the brain. By measuring the movement of water out of the central nervous system after abrupt changes in plasma osmolality, Fenstermacher and Johnson calculated the effective pore radius for the blood–brain barrier to be only 7 to 9 Å. This small pore size of the blood–brain barrier prevents movement not only of plasma proteins but also of sodium, chloride, and potassium ions between the intravascular compartment and the brain’s extracellular space ( Fig. 9.5 ). In effect, the blood–brain barrier acts like the semipermeable membrane of an osmometer, and movement of water across this membrane is determined by the relative concentrations of impermeable solutes.
This situation is markedly different in peripheral tissues, where endothelial cells do not form tight junctions and pore sizes in the capillaries may be as much as several orders of magnitude greater. Although these pores are small enough to preclude the movement of most protein components of plasma, electrolytes pass freely from the capillary lumen into the extracellular space. Thus, in peripheral tissues, movement of water between the intravascular space and the extravascular space is governed by the plasma concentration of large macromolecules (oncotic gradient) as defined by the Starling equation. In contrast, fluid moves in and out of the central nervous system according to the osmolar gradient (determined by relative concentrations of all osmotically active particles, including most electrolytes) between the plasma and the extracellular fluid. This difference in the determinants of fluid flux explains why the administration of large volumes of iso-osmolar crystalloid results in peripheral edema caused by dilutional reduction of plasma protein content, but does not increase brain water content or intracranial pressure (ICP).
There can be little doubt that osmolarity is the primary determinant of water movement across the intact blood–brain barrier. The administration of excess free water (either iatrogenically or as a result of psychogenic polydipsia) can result in an increased ICP and an edematous brain. Conversely, the intravenous administration of markedly hyperosmolar crystalloids (eg, mannitol) to increase plasma osmolarity results in a decrease in brain water content and ICP. Hyperosmolar solutions are used as standard therapeutic agents to treat intracranial hypertension.
In the presence of an intact blood–brain barrier, plasma osmolarity is the key determinant of water movement between the central nervous system and the intravascular space. However, what occurs when the brain is injured with disruption of the barrier? If the blood–brain barrier is partially disrupted, will blood vessels in the brain start to act more like peripheral capillaries? Experimental evidence is not conclusive, but if the injury is of sufficient severity to allow extravasation of plasma proteins into the interstitial space (ie, capillaries have become “leaky”), plasma oncotic pressure does not affect water movement, because no oncotic gradient between the plasma and the brain interstitial space can be produced (ie, the proteins leak out of the capillaries and into the brain tissue) ( Fig. 9.6 ). In an animal study using a cryogenic lesion as a model of acute brain injury, a 50% decrease in plasma oncotic pressure had no effect on regional water content or ICP. These results were confirmed in a subsequent study that demonstrated that reducing the plasma oncotic pressure from approximately 21 mmHg to 10 mmHg for 8 hours had no effect on ICP or brain water content in animals with a cryogenic brain injury despite the fact that the anticipated increase in water content was documented in peripheral tissues (muscle and jejunum).
Despite a lack of convincing experimental evidence that iso-osmolar crystalloids are detrimental, the neurosurgical literature is filled with admonitions to restrict the use of crystalloids in patients at risk for intracranial hypertension. In the case of the intact blood–brain barrier, neither theoretical nor experimental evidence suggests that colloids are more beneficial than crystalloids for either brain water content or ICP. The crystalloid-colloid question has been addressed in animal models of cerebral injury, with varying and sometimes conflicting results. Warner and Boehland studied the effects of hemodilution with either saline or 6% hetastarch in rats subjected to 10 minutes of severe forebrain ischemia. Despite an approximately 50% reduction in plasma oncotic pressure in the saline group (from 17.2 ± 0.8 to 9 ± 0.6 mmHg), no beneficial effect in terms of decreased edema formation was demonstrated in the hetastarch group. Similarly, in a study that used a cryogenic lesion as a model of cerebral injury, Zornow and colleagues found no differences in regional water content or ICP between animals that received saline, those that received 6% hetastarch, and those that received albumin.
In contrast, Korosue and associates found a smaller infarct volume and better neurologic status in dogs undergoing hemodilution with a colloid (LMW dextran) than in animals undergoing hemodilution with lactated Ringer’s solution after ligation of the middle cerebral artery. The researchers speculated (but did not provide evidence) that this beneficial effect was due to decreased edema formation in the ischemic zone. They further speculated that, in this model of moderate ischemic injury, the blood–brain barrier may become selectively permeable to ions with preservation of its impermeability to HMW compounds (eg, dextran and proteins). If this is the case, then the brain tissue in the ischemic region may act very much like tissues in the periphery (ie, decreases in plasma oncotic pressure result in increased water movement into the tissue). A study by Drummond and coworkers suggests that a similar situation may occur after traumatic brain injury (TBI). They subjected anesthetized rats to a 2.7 atm fluid percussion injury and then hemodilution with normal saline or a colloid. Brain water content was increased in the animals that received the normal saline. Thus, although the osmolality of the infused solution is the primary determinant of water movement between the vasculature and brain tissue in the noninjured state, apparently in cases of ischemic or traumatic injury, colloids may or may not be beneficial, depending on the severity and extent of the injury as well as the time at which brain water content is measured.
Beneficial effects of hypertonic solutions in cases of localized brain injury with disruption of the blood–brain barrier appear to be derived primarily from the ability of hypertonic solutions to cause a fluid flux out of brain tissue where the blood–brain barrier remains intact. In effect, the normal brain is dehydrated to compensate for the edema that forms in the vicinity of the lesion. The most likely mechanism for this beneficial effect is a decrease in brain water content in regions remote from the lesion.
Solutions for intravenous use
The anesthesiologist may choose from among a variety of fluids suitable for intravenous administration. These fluids may be categorized conveniently on the basis of osmolality, oncotic pressure, and dextrose content. The term crystalloid is commonly applied to solutions that do not contain HMW compounds and thus have an oncotic pressure of zero. Crystalloids may be hyperosmolar, hypo-osmolar, or iso-osmolar and may or may not contain dextrose. Some commonly used crystalloid solutions are listed in Table 9.1 . Crystalloids may be made hyperosmolar by the inclusion of electrolytes (eg, Na + and Cl − , as in hypertonic saline) or LMW solutes, such as mannitol (182 MW), glycerol (92 MW), glucose (180 MW), or urea (60 MW). Urea and glycerol are now rarely used because over time they penetrate the blood–brain barrier and may cause worsening of intracranial hypertension hours after their initial beneficial effect. Intravenous administration of mannitol has also been associated with the leakage into white matter near gliomas possibly due to the changes in blood–brain barrier permeability near tumors. Mannitol leak may exacerbate peritumoral edema and lead to ICP rebound.
The term colloid denotes solutions containing insoluble macromolecules, which do not pass through semipermeable membranes or capillary walls under normal conditions. Some colloid solutions have oncotic pressure similar to that of plasma (see Table 9.1 ). The examples of colloids are 6% hetastarch (Hespan), 5% and 25% albumin, the dextrans (40 and 70), and plasma. Dextran and hetastarch are dissolved in normal saline, so the osmolarity of the solution is approximately 290 to 310 mOsm/L with a sodium and chloride ion content of about 145 mEq/L.
Hyperosmolar Solutions
Hyperosmotic fluids have been successfully used as a resuscitation fluid in hemorrhagic hypovolemia as well as an osmotherapeutic agent reducing brain edema or elevated ICP. The reputed advantages of such solutions include a more rapid resuscitation with smaller infused volumes, improved cardiac output, decreased peripheral resistance, and lower ICP. The benefits of using a variety of hypertonic solutions in lowering ICP and improving cerebral blood flow have been demonstrated in a number of animal models. , Clearly, these solutions exert at least part of their beneficial effects by osmotically shifting water from the interstitial and intracellular spaces of the central nervous system to the intravascular space. Additional benefit may be derived from a reported reduction in cerebrospinal fluid production.
Although an acute beneficial effect has been demonstrated, the longer-term (24–48 hours) effect of such hyperosmotic fluid therapy remains unknown. One primary area of concern is the hypernatremia that results from many of these solutions. Although survival has been reported with serum sodium levels as high as 202 mEq/L, acute increases to values that exceed 170 mEq/L are likely to result in a depressed level of consciousness or seizures. Even with the administration of relatively small volumes (4.5 L) of moderately hypertonic saline (Na, 250 mEq/L; osmolarity, 514 mOsm/L), Shackford and coworkers , reported that serum sodium levels peaked at over 155 mEq/L in the postoperative period.
Several studies have reported a beneficial effect of hypertonic saline solutions in patients with intracranial hypertension. In one report, the administration of small volumes of markedly hypertonic saline in two patients resulted in a striking and sustained diminution in ICP. Both patients had suffered closed-head injuries with ICPs in the range of 30 to 50 mmHg. After conventional therapy with repeated doses of mannitol and hyperventilation had failed, 100 to 250 mmol of hypertonic saline was administered, resulting in prompt control of the intracranial hypertension. In a second study involving eight patients with a total of 20 episodes of intracranial hypertension that was resistant to standard forms of management (including hyperventilation and mannitol), 30 mL of 23.4% saline brought prompt and sustained decreases in ICP. In 80% of the cases, ICP dropped by more than 50% of the pretreatment value within 21 ± 10 minutes. Associated with this decrease in ICP was a significant rise in cerebral perfusion pressure from 64 ± 19 to 85 ± 18 mmHg 1 hour after hypertonic saline administration. In a controlled animal study designed to directly compare the cerebral effects of mannitol (20%) and hypertonic saline (3.2%), rabbits were randomly allocated to receive an equiosmolar load of one of these two solutions (10 mL/kg). Plasma osmolality increased to a similar extent in both groups, and no differences in ICP reduction or regional water content were identified ( Fig. 9.7 ).
Hypertonic saline can be administered as a bolus or continuous infusion and the dose can be titrated according to serum sodium levels and/or serum osmolality. , Frequent serum sodium checks every 4–6 hours are recommended when hypertonic saline is used as a continuous infusion or with repeated boluses. Another concern is the route of administration. Hypertonic saline infused via peripheral vein may lead to phlebitis. Alternatively central venous catheter placement may be required.
The possible benefit of adding furosemide to treatment with hypertonic solutions is controversial. In rats subjected to a closed-head injury, Mayzler and colleagues demonstrated an additional decrease in brain water content when furosemide was added to treatment with 3% hypertonic saline. However, this additional benefit was not seen when furosemide was added to treatment with mannitol. Although the protocols for these two studies differed in both the timing and the type of osmotic solutions administered, the findings suggest that the benefit of furosemide for a given patient is likely to be small, if it exists at all.
In a prospective controlled trial in children with head trauma, patients received a bolus (approximately 10 mL/kg) of either 0.9% or 3% saline. Baseline ICP values were similar in both groups (20 mmHg). No significant change in ICP was demonstrated in patients who received 0.9% saline. However, after a bolus of 3% saline, ICP decreased significantly and averaged 15.8 mmHg for the next 2 hours. In patients who received 3% saline, serum sodium concentrations increased from a mean of 147 mEq/L to 151 mEq/L.
Most recent clinical studies suggest that, compared to mannitol, hypertonic saline may achieve similar if not better brain relaxation conditions in patients undergoing craniotomy. Rozet and associates compared the effect of 5 mL/kg of 20% mannitol (1 g/kg) with that of an equiosmolar volume of 5 mL/kg of 3% hypertonic saline on brain relaxation in patients undergoing elective or urgent craniotomy for various neurosurgical procedures including tumor resection and cerebral aneurysm clipping. Both mannitol and hypertonic saline resulted in a similar increase in serum and CSF osmolalities and achieved a comparable degree of brain relaxation as assessed by the surgeon. Compared with 3% hypertonic saline, mannitol caused more diuresis ( P < .03) with less pronounced positive fluid balance at 3 hours (hypertonic saline group, 3230 ± 1543 mL; mannitol group, 1638 ± 1620 mL; P < .004); however, fluid balance values were not statistically different at 6 hours.
In a prospective randomized study, Dostal and associates compared the effects of intravenous mannitol and hypertonic saline on brain relaxation and postoperative complications in patients undergoing elective intracranial tumor surgery. Patients who received 3.75 mL/kg of 3.2% hypertonic saline had better brain relaxation conditions than those receiving 3.75 mL/kg equiosmolar 20% mannitol. Mannitol resulted in more pronounced diuresis, lower central venous pressures and natremia levels at the end of surgery. The authors did not find any significant differences in postoperative complications or lengths of hospital stay between the groups.
The studies by Rozet and Dostal suggest that hypertonic saline may be considered as an alternative to mannitol for brain relaxation in patients undergoing craniotomy, especially those who are hypovolemic or hemodynamically unstable. It must be noted, however, that both of these studies may have been insufficiently powered to allow direct comparison of adverse events, which are infrequent. Extensive review of Mortazavi and associates suggested that hypertonic saline offers better control of ICP than mannitol. The sodium load and consequent hypernatremia may be a concern in patients with neurologic injury who are at risk for seizures and who may have altered mental status due to an underlying injury.
In summary, hypertonic saline can exert a beneficial effect on intracranial hypertension while providing rapid volume resuscitation. It appears to be equivalent to mannitol. Whether it provides significant advantages over mannitol awaits further investigation.
Dextrose Solutions and Hyperglycemia
It is now fairly well established that hyperglycemia before or during ischemia worsens neurologic outcome. Several independent investigators using a variety of animal models have reached this conclusion. Worsened neurologic outcome has been repeatedly demonstrated after global ischemia of either the brain or the spinal cord. Elevations in plasma glucose do not have to be marked to produce a significant worsening of neurologic outcome. In an early study using a primate model, Lanier and associates showed that dextrose infusions (50 mL of 5% dextrose in 0.45% saline) markedly worsened the neurologic score after 17 minutes of global cerebral ischemia. Although plasma glucose levels were slightly higher in animals that received the dextrose infusion (181 ± 19 vs. 140 ± 6 mg/dL), this elevation did not even reach statistical significance. Drummond and Moore demonstrated a similar detrimental effect of hyperglycemia on spinal cord function after transient ischemia.
Although the apparent consensus is that hyperglycemia during transient global ischemia is detrimental, both beneficial and adverse effects of glucose have been shown in models of focal ischemia. Ginsberg and colleagues have demonstrated a decrease in infarct volume in rats made hyperglycemic before the ischemic event. Even greater beneficial effects (approximately 50% reduction in ischemic area) have been reported in a short-term study of hyperglycemia in cats after ligation of the middle cerebral artery. The significance of these findings is uncertain because in a long-term cat study (survival time of 14 days), the hyperglycemic animals sustained infarcts twice the size of normoglycemic controls.
The mechanism by which hyperglycemia worsens neurologic outcome is not clear. One hypothesis is that the glucose loading that occurs in central nervous system tissue during periods of hyperglycemia provides additional substrate for the production of lactic acid during the ischemic period. This increase in intracellular lactate is postulated to have a neurotoxic effect resulting in neuronal death. Although there is little doubt that lactate production increases in brains of hyperglycemic animals, the neurotoxic effect is less firmly established. Indeed, in neuronal cell cultures, lactic acidosis has been shown to be neuroprotective. , Another mechanism by which hyperglycemia may exacerbate neuronal injury is by enhancing glutamate release in the neocortex, but probably not in the hippocampus. ,
In clinical studies, hyperglycemia has been associated with worsened neurologic outcome and/or mortality after TBI, acute ischemic stroke (AIS), subarachnoid hemorrhage (SAH) and intracerebral hemorrhage (ICH). ,
Whether hyperglycemia directly causes worsened neurologic outcome or rather reflects the severity of tissue injury and its parallel stress response has been the subject of debate. , More recently, Schlenk showed that blood glucose levels > 140 mg/dL independently predicted unfavorable outcome and mortality at 12 months in patients with SAH besides age, Fisher and WFNS (World Federation of Neurosurgical Societies) grades. After adding a logistic regression model, authors stated that hyperglycemia > 140 mg/dL was independently predicting delayed ischemic neurological deficit. Cerebral glucose measured by microdialysis increased only at blood glucose levels higher than 140 mg/dL. The metabolic derangements, measured as lactate concentration and lactate/pyruvate ratio, were slightly elevated compared to patients with lower glucose levels but the difference was not statistically significant.
A post hoc analysis of data from the IHAST study showed that patients with glucose levels > 129 mg/dL at the time of aneurysm clipping had more cognitive impairment defined by neuropsychological function composite scoring at 3 months compared to normoglycemic patients. Patients with glucose > 152 mg/dL had significantly greater neurologic impairment defined by NIHSS (National Institute of Health Stroke Scale).
A recent retrospective observational study of Kurtz and associates evaluated the role of serum glucose variability in predicting cerebral metabolic distress and mortality. Plasma glucose variability was expressed daily as standard deviation (SD) of all serum glucose measurements per total monitoring period. Increased serum glucose variability SD of greater than 1.4 mmol/L (25.2 mg/dL) per day was associated with an increased risk of developing metabolic distress (lactate/pyruvate ration > 40) independently of GCS and brain glucose. Daily glucose SD greater than 1.4 mmol/L was also independently associated with hospital mortality. Davis and associates studied the effect of preoperative hyperglycemia on the risk of complications and length of ICU and hospital stay. Authors studied 918 craniotomy and spine-related neurosurgical cases and concluded that even mild preoperative hyperglycemia (> 120 mg/dL) was associated with an increased risk of a wide range of postoperative complications and prolonged length of stay.
The previously discussed studies show that hyperglycemia and glucose variability are associated with worse neurologic outcome, mortality, complication rate and increased hospital/ICU stay depending on the patient population studied. So, does treating hyperglycemia indeed make a difference in morbidity and mortality? A frequently cited study by Van den Berghe, demonstrating reduced morbidity and mortality in postoperative patients managed with “tight” (80–110 mg/dL) versus conventional (180–200 mg/dL) glucose control, stimulated interest in a more aggressive approach to treating hyperglycemia in the perioperative period. However, several subsequent studies examining intensive glucose control in broader ICU patient population raised concerns about the efficacy and safety of such an approach. In the NICE-SUGAR trial, 6104 ICU patients were randomized to undergo either intensive glucose control (target blood glucose levels 81–108 mg/dL) or conventional glucose control (target blood glucose levels ≤ 180 mg/dL). Mortality at 90 days was higher in the intensive-control group (27.5%) compared to the conventional-control group (24.9), and the treatment effect did not differ between medical and surgical patients. The incidence of severe hypoglycemia (glucose ≤ 40 mg/dL) was also higher in patients assigned to intensive glucose control. New single or multiple organ failure and hospital or ICU lengths of stay were no different between the groups. It is important to note that the median glucose level for intensive glucose control was 107 mg/dL vs. 142 mg/dL in conventional control, and the mean time-weighted blood glucose level 115 ± 18 vs. 144 ± 23 mg/dL, respectively.
Tight glucose control may carry a significant risk of hypoglycemic events and reduced survival, at least in the critically ill; and the question becomes how to minimize the very real risks associated with the treatment of hyperglycemia while maximizing the potential benefits. In many cases, the answer to this question is institution-specific and depends on the staffing and resources available to provide safe and effective care. Krinsely compared outcomes in 800 patients with intensive glucose management (target plasma glucose < 140 mg/dL) who were consecutively admitted to a medical-surgical ICU to outcomes in a historical control group of 800 ICU patients without standardized glycemic control. The incidence of hypoglycemia was similar in the two groups of patients. However, hospital mortality decreased 29.3% in the protocol group. Although the study was not adequately powered to allow a robust subgroup analysis, the decrease in mortality was especially marked in the neurologic patients. The weaknesses of this study are all those associated with nonrandomized clinical trials using historical controls.
Ooi and associates conducted a meta-analysis of studies reporting outcomes in neurological and neurosurgical patients and the role of glucose levels and insulin protocols. The authors compared intensive vs. conventional insulin therapy. Intensive insulin therapy involved intravenous administration of insulin via infusion pump for glucose target 80–110 mg/dL or looser; conventional therapy referred to subcutaneous insulin per sliding scale targeting glucose 180–220 mg/dL. The review showed that neurological and neurosurgical patients who received intensive insulin therapy had better neurological outcome and lower risk of infections than conventional therapy; mortality was not different. In contrast, Cinotti in his recent subgroup analysis of a randomized controlled CGAO-REA (Contrôle Glycémique Assisté par Ordinateur) study did not find that tight glycemic control improved 28-day mortality or neurologic recovery of patients with severe brain injury. It is important to note that the study evaluated neurological outcomes of patients with a variety of pathological states and mechanisms including TBI, SAH, ICH, cardiac arrest, brain tumor, brain abscess, and central nervous system infection.
Multiple studies have addressed glycemic control in aneurysmal SAH patients. Hyperglycemia on admission as well as during hospitalization has been associated with poor outcome. Some studies suggest that patients with SAH may benefit from stricter glycemic control than concluded by the NICE-SUGAR trial (< 180 mg/dL). Study of Latorre, which included 332 SAH patients from a prospective intensive care unit database, demonstrated that patients with good glycemic control managed by an aggressive hyperglycemia protocol targeting glucose levels 80–140 mg/dL resulted in reduction of poor neurological outcome at 3 and 6 months. Furthermore, a randomized prospective pilot trial on the effect of intensive insulin therapy in patients after intracranial aneurysm clipping with acute SAH showed reduced infection rates (27% vs. 42%, resp.) when glucose was maintained in a range of 80 to 120 mg/dL than a range of 80–220 mg/dL. However, the study was not intended to be sufficiently powered to address questions about the incidence of vasospasm, overall mortality, and neurologic outcome.
Treatment of hyperglycemia did not lead to improved outcome in a large multicenter randomized control trial of patients with acute stroke. In the GIST-UK trial, stroke patients who presented within 24 hours of symptoms onset and with initial plasma glucose 6–17 mmol/L (108–306 mg/dL) were randomized to either receive continuous infusion of variable dose insulin and potassium (GKI) to aim for capillary glucose level 4–7 mmol/L (72–126 mg/dL) or to receive normal saline infusion with no glycemic control for 24 hours. Excluded patient categories comprised those with SAH and insulin treated diabetes mellitus. The level of hyperglycemia on admission was moderate in the majority of patients, with mean level of 8.43 mmol/L (159 mg/mL). The study was stopped due to slow enrollment after entering 933 patients instead of the planned 2355 and hence was underpowered. The authors did not find difference in 90 day mortality or neurologic outcome between the study groups; however, a post hoc safety analysis showed increased mortality in those patients treated with GKI who had the greatest reductions in glucose levels (decrease of > 2 mmol/L).
In summary, hyperglycemia should be avoided in patients who are at risk for an ischemic event. Dextrose solutions should not be infused in the patient undergoing a neurosurgical procedure unless they are needed for the treatment or prevention of hypoglycemia. A more complex question is how to proceed when a patient enters the operating room with hyperglycemia. Although normalizing this patient’s plasma glucose level with insulin infusion is tempting, the effect of this intervention in reducing the risk of adverse outcome in a patient with neurologic injury is not clear.
The preceding evidence suggests that intensive glucose control (target < 110 mg/dL) carries a definite risk of hypoglycemia and may be harmful in the critically ill patient. The NICE-SUGAR trial set a reasonable glucose goal < 180 mg/dL in the general ICU patient population, with the caveat that the median glucose level in patients managed with conventional protocol was < 142 mg/dL. Bilotta’s review, focusing on glucose management in neurosurgical patients, leans toward the recommendation of moderate glucose management with the goal of 140–180 mg/dL. Evidence is accumulating, however, that tighter glucose control may improve neurologic outcome and lower risk of infections, especially in patients with SAH. , More prospective studies are needed to address optimal glucose management targets for patients with a specific type of neurologic injury or neurosurgical procedures.
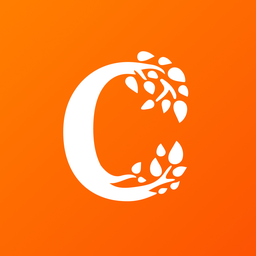
Full access? Get Clinical Tree
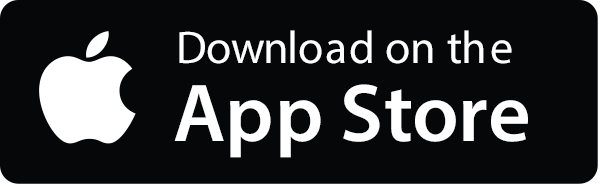
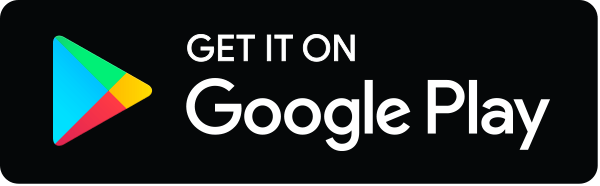