where Q is the net fluid flux (mL/min), (Pc − Pt) is hydrostatic pressure difference between capillary (c) and interstitium (i), and (πc − πi) is the oncotic pressure difference between the capillary and interstitium. Kf is the filtration coefficient for that membrane (mL/min/mmHg), and is the product of capillary surface area and capillary hydraulic conductance, and σ is the permeability factor (i.e., reflection coefficient) with one being impermeable, and zero being completely permeable.
The permeability factor explains why, in times of capillary leak as in shock states, colloids cannot maintain an oncotic pressure difference and tend to leak out into the interstitium. The general principle of fluid resuscitation is that intravascular hypovolemia should be replaced with isotonic fluid, which tends to distribute in the ECF (3:1) intravascular:interstitium. Hypotonic fluid will distribute between all body compartments with only a small amount remaining in the intravascular space (since water is freely permeable). Maintaining intravascular blood volume is of primary importance to deliver nutrition (via plasma) and oxygen (via red cells) to the tissues. Frequently used isotonic solutions are 0.9% normal saline (154 mEq/L of Na+ and Cl each) and lactated Ringer solution (130 mEq/L Na+, 109 mEq/L Cl−, 4 mEq/L K+, 3 mEq/L Ca2+, 28 mEq/L lactate).
The endpoint of fluid resuscitation continues to be an area of great debate since routine measurement of intravascular (plasma) volume is not the norm. Surrogate markers are used to assess adequate fluid resuscitation: blood pressure, heart rate, urine output, and parameters of perfusion and cardiac function. Although every clinician wants to treat patients to “euvolemia,” there are only a few centers measuring intravascular volume using radioisotope studies (see Blood Volume chapter). Debate will continue on how much fluid to give until an easy method of measuring intravascular volume is available.
DISORDERS OF WATER METABOLISM
Dysnatremias (hyponatremia or hypernatremia) are among the most common electrolyte disturbances and usually are associated with poor outcomes. This problem persists due to failure to promptly recognize a life-threatening condition and initiate appropriate treatment. This chapter will focus on the pathogenesis, diagnosis, treatment, and prevention of dysnatremias.
Regulation of Water Balance
ECF tonicity is generally reflected in the concentration of sodium in the serum. The serum sodium is proportional to the total body exchangeable sodium (Nae) plus the total body exchangeable potassium (Ke). This critical relationship is shown mathematically in Equation (1). Equation (1) is not used clinically; rather, it illustrates the relationship between total body solutes and total body water. Decreases in potassium often accompany hyponatremia, and replacement of intracellular solute losses can also be an important part of treating hyponatremia. As water intake and excretion are tightly regulated to maintain near-constant plasma osmolality (Fig. 134.1), disturbances in serum sodium indicate disorders in water balance, not gains or loss of sodium. This is a crucial point in understanding dysnatremias. The actions of ADH, also called arginine vasopressin (AVP) (1), on the kidney tightly regulates water excretion. To maintain water balance, an intact thirst mechanism and the ability of the kidneys to vary urinary concentration are required:
Renal Water Handling
The kidney can vary urinary concentration significantly and either excrete a large water load in very dilute urine or conserve water significantly such that the daily solute load is excreted in a small volume of urine. When the urinary filtrate passes into the cortical collecting duct, it is very dilute (as low as 50 mOsm/kg). As the urine moves through the cortical collecting duct into the collecting tubule, water reabsorption occurs in the presence of ADH. In the absence of ADH activity (as in diabetes insipidus), urine concentration will remain very low (50 to 80 mOsm/kg). When ADH activity is maximal, urinary concentration can be as high as 1,200 mOsm/kg. This ability to excrete very dilute or a very concentrated urine allows the body to achieve water balance across a very wide range of water intake (between approximately 0.8 and 15 L/d). Impairments of this hormonal system that links perturbations in serum osmolality as detected by osmoreceptors in the hypothalamus to variations in urinary concentration can lead to impairments in water balance. Administration of water to a patient with impaired water excretion (Table 134.1) can lead to hyponatremia.
TABLE 134.1 States of Impaired Water Excretion in the ICU Resulting in Hyponatremia |
![]() |
Electrolyte Free Water
Electrolyte free water is a useful concept in the approach to the patient with a disturbance in water balance. Electrolyte free water is a conceptual volume of a body fluid (usually urine) that represents the volume of that fluid that would be required to dilute the electrolytes contained within total volume of the fluid to the same tonicity as plasma electrolytes (Fig. 134.2). The remainder of the volume (total volume minus electrolyte free water) can be thought of as containing the nonelectrolyte osmoles. This nonelectrolyte water excretion is the amount of water excreted above the excretion of electrolyte solutes, and thus if it is not replaced, will have an effect on the plasma sodium concentration. In other words, the osmolality of a solution is not important in determining if it contains “free water”; rather, it is the concentration of electrolytes that is important. An example of this is that the administration of dextrose solutions provides the same amount of free water as an equal volume of deionized water, whereas 0.45% normal saline contains approximately 50% less. Electrolyte free water clearance can be calculated as a convenient clinical tool in assessing water need in a patient. The amount of electrolyte free water in a body fluid (e.g., urine, sweat, nasogastric [NG] aspirate) is calculated by the following formula:

FIGURE 134.1 Water intake and excretion regulation. ADH, antidiuretic hormone.

FIGURE 134.2 Electrolyte free water.
Clinical Application of Electrolyte Free Water Clearance
The most important conceptual point to understand is that the urine electrolytes and not the urine osmolality determine the degree of free water excretion in the urine. It is not always necessary to calculate an exact value for the electrolyte free water clearance if the relationship between the plasma electrolytes and the urine electrolytes is understood. If the concentration of electrolytes in the urine is greater than the concentration of electrolytes in the plasma, then free water is not being excreted in the urine. If the concentration of electrolytes in the urine is less than that in the plasma, then the patient is excreting free water in the urine. This relationship is illustrated in Figure 134.3. This is a simple test that can allow for a quick assessment of the ongoing losses of water in the urine.
TABLE 134.2 Nonosmotic Causes of Arginine Vasopressin Excess (81) |
![]() |
HYPONATREMIA
Hyponatremia commonly occurs in hospital settings and especially in the ICU setting (Table 134.2). Often the condition is minimally symptomatic, but hyponatremic encephalopathy (brain dysfunction due to cerebral edema in turn due to hyponatremia) can result (2–4). Hyponatremic encephalopathy is a life-threatening medical emergency, and it must be recognized and promptly treated as it can often lead to death or devastating neurologic complications (5,6). Differentiating between these two spectrums of the disease presentation is critical. Among risk factors for life-threatening hyponatremia are female gender of premenopausal age (7), children (5), and hypoxia (7). Research over the last two decades has elucidated the pathogenetic mechanisms that underlie these risk factors, and this has prompted new thinking and mandated shifts in the clinical approach to hyponatremia.
Pathogenesis
Hyponatremia is defined as a serum sodium below 135 mEq/L. The ability of the kidney to dilute the urine and thus excrete free water is the body’s primary defense against the development of hyponatremia. Excess ingestion of water as the sole cause of hyponatremia is rare since the typical adult with normal renal function can excrete a massive free water load (15 L of free water per day). The combination of factors necessary for the development of hyponatremia are free water intake in the setting of an underlying condition that impairs free water excretion (see Table 134.1). The states that impair water excretion are usually states where ADH release is a physiologic response to a stimulus such as volume depletion, pain, nausea, postoperative state, or congestive heart failure (due to decreased circulating blood volume). In other instances, pathologic release of ADH occurs in syndrome of inappropriate ADH release (SIADH) and with certain medications such as thiazide diuretics and anticonvulsants.

FIGURE 134.3 Relationship of electrolyte concentration in urine and plasma to the amount of free water excreted.
Brain Defenses against Cerebral Edema
Hyponatremia induces an osmotic gradient that favors water movement into the brain leading to cerebral edema and neurologic injury. However, the brain is contained within a specialized compartment separated from the systemic circulation by the blood–brain barrier that impedes entry of water and has specialized mechanisms for handling water fluxes (8–10). The blood–brain barrier is a specialized structure with tight junctions between vascular endothelial cells (11–13) that interface with glial cells (astrocytes) on the brain side of the blood–brain barrier. Astrocytes form an important part of the microvascular compartment in the brain and project foot processes that abut the endothelial cells of the brain capillaries (14). This highly specialized cell performs many supporting functions in maintaining the fluid environment and the electrolyte milieu of the extracellular space of the brain (15,16). Among these functions is shunting of potassium from the microenvironment by uptake and release of potassium with water accompanying, in the perivascular space away from neurons. This function is accomplished through a concentration of aquaporin-4 water channels and Kir4.1 potassium channel located at the end-feet around the perivascular space (8). There is increasing evidence that glial cells also have an important role in brain water handling. The observation that glial cells (but not neurons) selectively swell following hypotonic stress presaged the existence of a glial-specific water pore, which has now been shown to be aquaporin 4. Mice lacking aquaporin 4 do not develop cerebral edema in response to hyponatremia, suggesting that these channels may have an important role not only in normal water regulation in the brain, but also in the pathogenesis of hyponatremia-induced cerebral edema (17). During states of cytotoxic brain edema, water is shunted through the astrocyte, which swells, and the neuron is protected from this influx of water. Therefore, astrocytes are the principal regulator of the brain water content as they comprise the bulk of the intracellular space, and the response of these cells following osmolar stress is an important determinant of the changes in brain volume during hyponatremia. This swelling of astrocytes is a principal factor in the development of cerebral edema during hyponatremic stress (Fig. 134.4).
The brain has several defenses against the development of cerebral edema. The first response is the shunting of cerebrospinal fluid (CSF) from within the brain, but this mechanism has a limited capacity to buffer volume changes (18). Immediately after a volume stress, cell volume regulatory mechanisms in the cerebral astrocytes play an important role in reducing brain volume through reduction in cellular osmolyte (mainly electrolyte) content. These are adaptive mechanisms that are used by multiple cell types to counteract an increase in cell volume; however, the astrocyte responds to cellular swelling differently than many other cells (18). In erythrocytes, white blood cells, and epithelial cells, swelling occurs due to a hypotonic environment and calcium influx that begins a series of events termed the regulatory volume decrease (RVD) mediated by activation of K+ and Cl− channels that allow these ions to be released into the extracellular environment. In glial cells this is not the predominant response. The glial cell uses ATP-dependent mechanisms (18) that require the Na+/K+ ATPase during which ions are extruded from the glial cell and water obligatorily follows the extruded ions, reducing brain volume and protecting from the development of cerebral edema. This response is ongoing, and in animal models of acute hyponatremia, brain water content is close to the baseline value 6 hours after induction of acute hyponatremia (19). The Na+/K+ ATPase is ubiquitous and plays an essential role in cellular ion homeostasis. In the brain, this enzyme is very important in the response of the cell to volume stress and hypotonic insult (20). The enzyme has binding domains for sodium, potassium, and cardiac glycosides and requires the hydrolysis of ATP to ADP to provide energy for moving these ions against concentration gradients (21). Therefore, in vitro evidence suggests that the actions of the sodium potassium ATPase are the important immediate responses in determining the brain’s response to hypo-osmolar stress.

FIGURE 134.4 The pathogenesis of cerebral edema following hyponatremic stress. A: Aquaporin-4 (depicted in blue) expressed on the astrocyte foot process form an important component of the blood–brain barrier. B: During hyponatremic states, intracellular osmolality of the astrocytes exceeds that of the plasma and water follows it concentration gradient, through aquaporin-4 channels into the astrocytes leading to cerebral edema. (Courtesy of Sydney Achinger.)
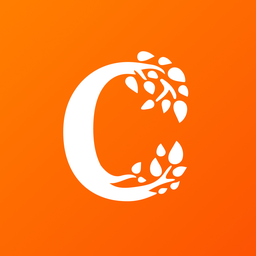
Full access? Get Clinical Tree
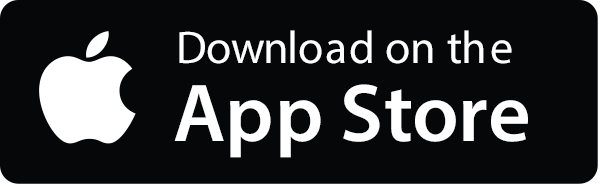
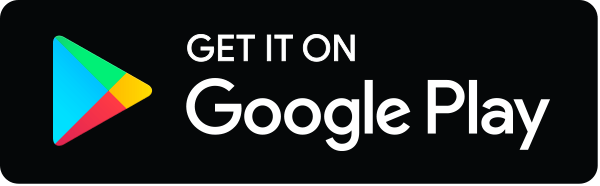