Abstract
Fetal life in utero differs significantly from postnatal life. The fetus relies completely on the mother and the placenta for basic metabolic needs such as nutrient delivery, gas exchange, acid-base balance, and electrolyte homeostasis. During gestation, the fetus gradually assumes the responsibility for many of the vital physiologic functions that must be assumed after the abrupt transition to physiologic independence at birth. Knowledge of fetal physiology, and the timing associated with these developmental changes, is necessary for the optimal provision of analgesia and anesthesia during pregnancy and childbirth.
Keywords
Fetus, Physiology, Development, Metabolism, Fetal systems
Chapter Outline
Fetal Environment, 77
Amniotic Fluid, 77
Oxygen Supply and Transport, 78
Glucose and Lactate Metabolism, 79
Amino Acid and Lipid Metabolism, 80
Thermoregulation, 80
Fetal Cardiovascular System, 81
Circulatory Pattern, 81
Blood Volume, 81
Cardiac Development, 82
Cardiac Output and Distribution, 82
Fetal Pulmonary System, 84
Fetal Renal System, 85
Fetal Hematologic System, 85
Fetal Gastrointestinal System, 86
Fetal Nervous System, 87
Structural and Functional Brain Development, 87
Cerebral Metabolism, 88
Cerebral Blood Flow, 88
Nociception, 88
Fetal life in utero differs significantly from postnatal life. The fetus relies completely on the mother and the placenta for basic metabolic needs such as nutrient delivery, gas exchange, acid-base balance, and electrolyte homeostasis. During gestation, the fetus gradually assumes the responsibility for many of the vital physiologic functions that must be assumed after the abrupt transition to physiologic independence at birth. Knowledge of fetal physiology, and the timing associated with these developmental changes, is necessary for the optimal provision of analgesia and anesthesia during pregnancy and childbirth.
Fetal Environment
Amniotic Fluid
The fetus is surrounded by amniotic fluid, a complex fluid that changes as the pregnancy progresses. Amniotic fluid serves a number of vital roles, including the facilitation of fetal growth, the provision of a microgravity environment that cushions the fetus, and the generation of a defense mechanism against invading microbes. The formation and maintenance of amniotic fluid is an intricate process that depends on fetal maturation and maternal hydration, hormonal status, and uteroplacental perfusion.
Amniotic fluid during early embryogenesis is principally derived from maternal plasma by the passage of water and solutes through aquaporin water channels expressed in the fetal maternal membranes (amnion and chorion). The expression of aquaporins changes as gestation advances and with certain pathologic states, such as polyhydramnios. Between 10 and 20 weeks’ gestation, the volume of amniotic fluid increases in a predictable and linear manner from approximately 25 mL to 400 mL. During this period, the composition of amniotic fluid is similar to fetal extracellular fluid, owing to the absence of keratin in the fetal skin. After this period, the volume of amniotic fluid is a function of production, from fetal urine (600 to 1200 mL/day near term) and respiratory tract secretions (60 to 100 mL/kg fetal body weight/day), and removal through fetal swallowing (200 to 250 mL/kg fetal body weight/day). Amniotic fluid volume is also influenced by intramembranous (between amniotic fluid and fetal blood within the placenta) and transmembranous (between amniotic fluid and maternal blood within the uterus) pathways in both physiologic and pathophysiologic states. Finally, the status of maternal hydration and the amount of decidual prolactin may alter the transfer of amniotic fluid through fetal and maternal tissues. Amniotic fluid volume plateaus at 800 mL at around 28 weeks’ gestation, after which it declines to approximately 400 mL at term.
The composition of amniotic fluid undergoes more marked variation than its volume. During the first trimester, amniotic fluid consists mostly of water and electrolytes and contains minimal protein. Keratinization of the fetal skin is complete by 25 weeks’ gestation and decreases the permeability of fetal tissues to water and solutes. The impact of this process, coupled with the ability of the fetal kidneys to produce urine, results in increased amniotic fluid concentrations of urea and creatinine, decreased concentrations of sodium and chloride, and reduced osmolality. By term, the osmolality of amniotic fluid is about 85% to 90% that of maternal serum. A variety of carbohydrates, proteins, lipids, electrolytes, enzymes, and hormones, which vary in concentration depending on the gestational age, are also present; some of these elements, particularly the amino acids taurine, glutamine, and arginine, serve a nutritive function for mitotic cells involved in trophoblastic growth and placental angiogenesis. An abundance of growth factors are found in amniotic fluid, including epidermal growth factor, transforming growth factor-alpha, transforming growth factor-beta 1, insulin-like growth factor-1, erythropoietin, and granulocyte colony-stimulating factor; many of these growth factors play an important role in fetal intestinal development.
Antimicrobial defenses within the amniotic fluid are primarily composed of humoral mediators such as alpha-defensins, which are released from neutrophils, especially in the setting of preterm labor and/or chorioamnionitis. Other humoral mediators include lactoferrin, calprotectin, leukocyte protease inhibitor, and cathelicidin, which have significant activity against bacteria, viruses, and fungi. Cellular mediators of the immune response are poorly characterized in amniotic fluid, and it remains unclear if the macrophages that are present serve a scavenging or an antimicrobial role. Neutrophils are usually absent from the amniotic fluid of a healthy fetus, and their presence typically signifies an inflammatory or infectious process.
Biochemical and cellular analyses of amniotic fluid provide valuable information on chromosomal abnormalities, neural tube defects, prenatal infections, and most inborn errors of metabolism. Several amniotic fluid–based indices, including the lecithin-sphingomyelin ratio, the phosphatidylglycerol level, lamellar body count, surfactant-to-albumin ratio, and electrical conductivity, are commonly used to assess fetal lung maturity. Bilirubin levels can be determined by measuring the optical density of amniotic fluid, which assists in the monitoring of fetal hemolysis. Estimation of the amniotic fluid levels of S100-beta (a protein released from injured astrocytes) and cell-free fetal nucleic acids may serve as early screening tests for perinatal neurologic damage and fetal development, respectively. Finally, amniotic fluid is a valuable reservoir for cell types of multiple lineages at different maturational ages; approximately 1% of these cells are pluripotent, thereby representing a novel source of stem cells.
Oxygen Supply and Transport
The fetus has almost no oxygen reserve and depends on maternal sources of oxygen delivery. Acute hypoxia can have immediate, severe consequences, such as perinatal death, hypoxic encephalopathy, and cerebral palsy. Chronic reductions of oxygen supply often lead to fetal growth restriction and may have long-term consequences for brain, heart, and kidney function through epigenetic changes. Hypoxia-inducible factors (HIFs) act on specific signaling pathways that modify target gene expression. When hypoxia occurs during critical periods of heart development, apoptosis of cardiomyocytes can result, leading to a myocardium that is less resilient to ischemic insults in later life. In addition, chronic hypoxia can influence fetal development of brain and kidney function and metabolism, subsequently leading to problems in adulthood.
Oxygen is an essential substrate for cell survival, because it serves as the final electron acceptor in the electron transport chain. When oxygen is scarce, the electron transport chain is compromised, resulting in decreased oxidative phosphorylation and adenosine triphosphate (ATP) production. Hypoxia ensues when the demand for oxygen exceeds the available supply. In adult tissues, hypoxia occurs at oxygen tensions < 20 mm Hg (2.7 kPa) (normal, 40 mm Hg [5.3 kPa]). By contrast, in fetal tissues, hypoxia occurs at oxygen tensions < 17 mm Hg (2.3 kPa) (normal, 20 to 25 mm Hg [2.7 to 3.3 kPa]). This implies that the environment for fetal development exhibits a smaller margin of safety before reaching a state of oxygen insufficiency and highlights the importance of maintaining adequate uteroplacental perfusion and fetal cardiac output to ensure fetal oxygen delivery. Ultimately, oxygenation of fetal tissues depends principally on the partial pressure of oxygen gradient between maternal and fetal blood and the different types of hemoglobin that exist in maternal and fetal blood.
Placental oxygen concentrations change with gestation. In early pregnancy, the placental intervillous space is free of maternal blood cells, thereby requiring the embryo to rely on endometrial secretions and maternal plasma for its energy requirements. The first trimester placenta has (1) an oxygen partial pressure (P o 2 ) of approximately 20 mm Hg (2.7 kPa); (2) only a few capillaries, which are located mainly in the center of the mesenchymal core; and (3) a trophoblastic layer that is twice the thickness of that in the second trimester. Moreover, the fetal red blood cells are nucleated, and the exocoelomic cavity contains antioxidant molecules instead of an oxygen transport system. These anatomic and physiologic features, which limit the transfer of oxygen and the creation of free radicals, protect the highly sensitive embryo from the effects of oxidative stress and keep the embryonic cells in their pluripotent state. At the end of the first trimester, an exponential increase in fetal growth creates significant demands for oxygen and nutrients ( Fig. 5.1 ). In response, cytotrophoblastic cells interact with the smooth muscle of maternal spiral arteries, resulting in vessel dilation and the provision of oxygen-rich maternal blood flow to the placenta ( Fig. 5.2 ).


The placenta acts as both a conduit and consumer of oxygen. The placenta is metabolically active and performs important roles in carbohydrate and amino acid metabolism, protein synthesis, and substrate transport. Almost 40% of the oxygen delivered to the pregnant uterus is needed to support the metabolic processes of the placenta. During periods of hypoxia, the placenta appears to alter its metabolism to diminish its consumption of oxygen, most likely by increasing glycolysis. This process can maintain fetal oxygen supply but, if hypoxia is ongoing, fetal growth restriction (also known as intrauterine growth restriction) may result. When the oxygen supply is compromised, the fetus shunts blood flow from peripheral tissues to vital organs (see later discussion), converts to greater use of anaerobic pathways, and induces gene expression that enables improved survival in a low-oxygen environment. The presence of fetal hemoglobin (hemoglobin F), with its higher concentration (approximately 18 g/dL) and greater affinity for oxygen than adult hemoglobin (see later discussion), results in a fetal arterial blood oxygen content that is only marginally lower than that in the adult, despite a lower oxygen tension.
Glucose and Lactate Metabolism
Glucose is the primary substrate for energy production in the fetus. Under normal conditions, gluconeogenesis is absent in mammalian fetuses; the only source of glucose is that which is transferred across the placenta. Glucose uptake into fetal tissues is regulated by glucose transporters (GLUT) of which the expression increases or decreases in response to acute and chronic changes in fetal glucose concentration. Fetal glucose concentration is linearly related to maternal concentration over a range of 3 to 5 mmol/L (54 to 90 mg/dL; Fig. 5.3 ); studies in isolated placentas suggest that this relationship continues up to a glucose concentration of 20 mmol/L (360 mg/dL). The placenta uses the majority of glucose delivered to the uterus for oxidation, glycogen storage, and conversion to lactate, with the remainder being transferred to the umbilical venous blood by facilitated, carrier-mediated diffusion. The amount of glucose supplied to the fetus appears more than adequate during normal conditions; ovine uterine blood flow must be reduced by greater than 50% before a decrease in fetal glucose uptake or fetal arterial glucose concentration is observed.

The umbilical cord blood glucose uptake is approximately 5 mg/kg/min at normal maternal arterial plasma glucose concentrations. Because the umbilical glucose/oxygen quotient varies from approximately 0.5 in sheep to 0.8 in human fetuses during labor, it is assumed that substrates other than glucose are used to support fetal oxidative metabolism; it is estimated that lactate and amino acids provide approximately 25% each of the total fetal energy requirements.
Lactate is produced even in well-oxygenated fetal lambs, with total lactate production being approximately 4 mg/kg/min. Although the exact origin of fetal lactate is unclear, skeletal muscles and bones have been identified as sources of lactate production under resting conditions. Lactate production increases during episodes of acute hypoxemia, although this response may be blunted in fetuses previously exposed to oxidative stress. Lactate consumption occurs in the fetal myocardium and liver. Short-term exogenous lactate infusion in fetal lambs (sufficient to lower the pH to 7.20) results in transient fetal bradycardia and increased fetal breathing movements but no other adverse effects.
Amino Acid and Lipid Metabolism
The fetus uses amino acids for protein synthesis, growth, and oxidation. Most maternal-to-fetal amino acid transfer occurs against a concentration gradient and involves energy-dependent transfer mechanisms. Under conditions in which fetal aerobic metabolism is decreased, amino acid uptake by the placenta and fetus may be reduced because it involves an expenditure of energy. Hypoxia results in a large reduction in nitrogen uptake in fetal lambs. During maternal fasting, fetal amino acid uptake does not change; however, enhanced fetal proteolysis may occur, which subsequently results in amino acid oxidation or gluconeogenesis.
Lipid products are transferred from the mother to the fetus. The fetus requires free fatty acids for growth, brain development, and the deposition of body fat for postnatal life. Fatty acids are transferred across the placenta by simple diffusion. Ketones are also transferred by simple diffusion; in humans, the maternal/fetal ketone ratio is approximately 2.0. The fetus can use ketones as lipogenic substrates or as energy substrates in the brain, kidney, heart, liver, and placenta. Beta-hydroxybutyrate (fatty acid) metabolism can occur in the placenta, brain, and liver during episodes of fetal hypoglycemia that result from maternal fasting. Cholesterol synthesis or free cholesterol diffusion does not appear to occur in the placenta. However, there is a significant correlation between maternal and fetal concentrations of lipoprotein(a), implying that diffusion of lipoprotein(a) may occur.
Thermoregulation
Intrauterine fetal temperature largely depends on maternal temperature. However, owing to the high metabolic rate in the fetus, the net flow of heat is from the fetus to the mother. Compared with the mother during the third trimester, the fetus produces approximately twice as much heat (on a weight-adjusted basis) and maintains a temperature 0.5° C higher. This maternal-fetal difference in temperature remains relatively constant and is referred to as the “heat clamp.”
The placental circulation is responsible for approximately 85% of the heat exchange between the mother and fetus. The remaining 15% is dissipated through the fetal skin and transferred through the amniotic fluid and the uterine wall to the maternal abdomen. As a consequence, fetal temperature may be rapidly affected by changes in umbilical blood flow; fetal temperatures rise quickly on occlusion of umbilical blood flow in both baboons and sheep. In humans, fetal temperatures increase during uterine contractions, which may be a result of intermittent obstruction of umbilical cord blood flow. Whether this rise in fetal temperature contributes to acute hypoxic-ischemic brain damage in the setting of umbilical cord prolapse is currently unknown. However, relatively small increases in temperature increase the sensitivity of the fetal brain to hypoxic injury.
The fetus generates heat through high metabolic activity, as thermogenic mechanisms are not developed until the end of gestation and thus largely inactive in utero. Newborns are at high risk for rapid heat loss caused by amniotic fluid evaporation and a sudden decrease in ambient temperature. Because of their small muscle mass, neonates are incapable of significant heat production through shivering; thus nonshivering thermogenesis plays an important role in maintaining temperature. Nonshivering thermogenesis occurs in brown adipose tissue, which is unique from other adipocytes owing to the significant presence of mitochondria, fat vacuoles, sympathetic innervation, and blood vessels. In the mitochondria of brown adipose tissue, ATP production is uncoupled from the oxidative process, resulting in an increase in heat production and oxygen consumption. Nonshivering thermogenesis is inhibited in utero, most likely owing to the presence of adenosine and prostaglandin E 2 , which have strong antilipolytic actions on brown tissue, inadequate oxygen levels, and low levels of intrauterine catecholamines and thyroid hormones. The inhibition of nonshivering thermogenesis is believed to be beneficial to the fetus, in that it allows for conservation of oxygen and accumulation of brown adipose tissue.
Fetal Cardiovascular System
The cardiovascular system is one of the first functional organ systems in the developing fetus. The morphologic development of the human heart, from its first appearance as a heart tube to its development as a four-chambered structure, occurs between 20 and 44 days’ gestation. Even before the development of the four-chambered heart, the valveless heart tube generates unidirectional flow, typically around 21 days’ gestation.
Circulatory Pattern
Fetal circulation differs significantly from the postnatal circulation. The fetal cardiovascular system is anatomically arranged in such a way as to allow blood to bypass the lungs and provide maximal perfusion of the placenta, where gas and nutrient exchange occur. The fetal systemic circulation receives cardiac output from both the left and the right ventricle, with the ventricles working in parallel. In contrast, during postnatal life, the left and right circulations are separated and the ventricles work in series.
Fetal blood flow is characterized by three anatomic communications between the left and right circulations: the ductus venosus, the foramen ovale, and the ductus arteriosus ( Fig. 5.4 ). Oxygenated blood travels from the placenta through the umbilical vein to the ductus venosus, which connects the umbilical vein with the inferior vena cava, thus bypassing the portal circulation and the liver. At mid-gestation, approximately 30% of the umbilical venous blood is shunted through the ductus venosus; from 30 to 40 weeks’ gestation, this fraction decreases to approximately 20%, although a significant increase can occur in response to hypoxia (see later discussion). Once in the right atrium, oxygenated blood preferentially flows through the foramen ovale to the left atrium and left ventricle, before entering the aorta and the systemic circulation. This mechanism ensures the delivery of well-oxygenated blood to the brain and the heart, which are the two organs with the highest oxygen requirements. The preferential shunting of ductus venosus blood through the foramen ovale into the left atrium is related to the umbilical venous pressure and the portocaval pressure gradient.
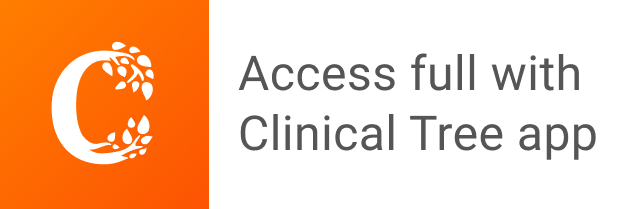