Definition and History
Extracorporeal life support (ECLS) is defined as total or partial diversion of a patient’s circulating blood volume into a device that can act as a “lung,” providing oxygenation and carbon dioxide removal, and a pump, which can act like a “heart,” providing circulatory support, or a combination of both functions for patients suffering from both cardiac and pulmonary failure. Adapted from heart-lung machines used for cardiopulmonary bypass, this temporary form of support was termed extracorporeal membrane oxygenation (ECMO) in the past.1–4 Because of vast changes in the circulatory devices now available and the patient populations being treated, however, the term ECMO is being replaced by ECLS as the designation for extracorporeal life support of a variety of indications and devices. Both are used interchangeably in the chapter. Figure 20-1 shows an example of an early cardiopulmonary bypass circuit.
ECLS was first developed to provide respiratory support in premature infants who had inadequate lung development to support gas exchange compatible with life. Difficulties with intracranial hemorrhage secondary to the heparinization required to prevent clotting of the artificial membrane lung and the circuit, however, caused ECLS to quickly fall out of favor in this population. Work continued, and following the development of the silicone-membrane oxygenator by Kolobow and others, ECLS was successfully applied to term newborns with respiratory failure.5,6 One of the first of these patients was a newborn named Esperanza (which means hope) by the neonatal intensive care unit nursing staff who were caring for her at the University of California at Irvine in 1976. Esperanza had hypoxemia secondary to what we now would call persistent pulmonary circulation of the newborn. Dr. Robert Bartlett and colleagues were doing research on ECMO at the University of California at Irvine and were contacted for assistance with Esperanza. Their laboratory device was quickly approved by the institutional review board for emergency use, and adapted and applied in an attempt to save Esperanza. The procedure worked well and Esperanza is now a grown woman with children of her own. Following this event, other centers reported success in the use of ECLS in newborns with respiratory failure from diseases such as meconium aspiration syndrome, persistent pulmonary hypertension, and congenital diaphragmatic hernia.7–10
This initial success was met with some skepticism, and a randomized controlled trial of ECMO or ECLS versus conventional ventilation was undertaken in newborns with persistent pulmonary hypertension in the late 1980s. This was a two-phase project: if excessive mortality or benefit was noted in one group, the next twenty patients would be treated with the more successful therapy.11 The safety monitoring board stopped Phase 1 of the study when four of the ten patients in the conventional arm died while all nine of the patients in the ECMO arm survived. Of the next twenty patients (all receiving ECMO), nineteen patients survived. Thus, 97% (twenty-eight of twenty-nine) of the ECMO patients and 60% (six of ten) of the conventional ventilator patients survived (p < 0.05). This trial aroused much controversy, especially regarding the randomization scheme and the fact that only families of patients randomized to ECMO were asked for consent.12 Another randomized study from the United Kingdom also favored ECLS in neonatal respiratory failure.13 Today, ECLS is an accepted therapy for neonates with refractory respiratory failure.14
At the same time, adults with respiratory failure were also receiving ECLS.15,16 One of the first case reports, by Hill in 1972, involved a young man who suffered injuries, including a ruptured aorta, as the result of a motorcycle accident. Supported by a cumbersome ECMO circuit for 3 days, the man survived.17 Further case reports and a historic meeting regarding ECLS held in Copenhagen in the late 1970s led to a National Institutes of Health-sponsored trial of ECMO versus conventional therapy in adult respiratory failure. Published in 1979 in the Journal of the American Medical Association (JAMA), the ECMO and conventional therapy groups had equivalently poor survival (<10%), and major complications of bleeding.18 The study also took place during an epidemic of influenza, which may have skewed results. The study, in retrospect, was flawed given lack of consensus definitions of conventional treatment, maintenance of high levels of mechanical ventilator support in both groups with no attempt at lung-protective ventilation in the ECMO patients, and imprecise definitions of organ failure. Of a planned 300-patient enrollment, the study was stopped at ninety-two patients because of the high mortality rates in both groups.
These dismal results dampened enthusiasm for ECLS in the adult patient and it became poorly accepted among adult clinicians in the United States. Across the globe, however, investigators in Italy, Sweden, Germany, Japan, and elsewhere continued work on supporting adult respiratory failure with ECLS techniques.19 Research by Gattinoni added to the understanding of the pathophysiology of adult respiratory failure, the detrimental impact of mechanical ventilation at high levels on the lung and the potential protective role of ECLS in lung recovery.20 When Gattinoni published results of a low positive pressure ventilation scheme combined with ECLS, primarily for carbon dioxide removal, which achieved 49% survival in patients with similar entry criteria as the failed National Institutes of Health trial, interest in adult ECLS again began to arise. Further studies with similar results from other centers began to appear and culminated with a conference on ECLS in Marburg, Germany, in 1988.21,22
As a means of sharing information regarding patients receiving ECLS, an informal gathering of clinicians, bench researchers, industry representatives, technicians, and survivors was held in 1989. Titled the Extracorporeal Life Support Organization (ELSO), this volunteer consortium has established an international database that contains information on more than 45,000 patients from more than 100 centers. The annual symposium continues to provide dissemination of technology, networking, and discussions of advancements and areas of needed work in the field, and provides an important forum for interaction between clinicians, technicians, and industry and bench researchers.23,24 ELSO has also established practice guidelines and standards of care for patients receiving ECLS. Membership in ELSO gives access to database information for research or center inquiry for specific patient populations. Quarterly reports on both individual and collective center outcomes, complications, equipment, and other parameters are provided. ELSO also provides small levels of grant support to advance the field of ECLS. Centers providing ECLS who do not report results to the ELSO group weaken the ability to track ECLS changes and outcomes globally. Currently undergoing some revision, to refine severity of illness parameters and other specifics, the ELSO registry continues to provide the most comprehensive information regarding trends in ECLS on an ongoing basis. Further information regarding this group can be found at www.ELSO.med.umich.edu.
As with other groups, use of ECLS in pediatric respiratory failure patients has been controversial. Attempts to establish mortality risks and outcome in pediatric respiratory failure were a prelude to a randomized trial of ECLS versus conventional care.25 The earlier report of Timmons et al on 470 patients from forty-one pediatric intensive care units with respiratory failure (defined as a fractional inspired oxygen concentration [FIO2] ≥0.50 and positive end-expiratory pressure [PEEP] ≥6 cm H2O for ≥12 hours) noted a mortality rate of 43%.26 Using variables that included age, operative status, Pediatric Risk of Mortality (PRISM) score, FIO2, respiratory rate, peak inspiratory pressure, PEEP, arterial oxygen tension (PaO2), and arterial carbon dioxide tension (PaCO2), a pediatric respiratory failure score was developed and validated against another patient subset.27 This led to attempts to perform a randomized controlled trial of ECMO in pediatric respiratory failure patients using pediatric respiratory failure score guidelines. The trial was stopped early when interim analysis revealed a mortality of 18% in the control group (contrasted with a predicted mortality of 36% from earlier evaluations), and the likelihood of achieving statistical significance between ECMO and control groups was deemed futile. This trial was also likely impacted by changes that occurred in the management of respiratory failure: positive inspiratory pressure in the historical group (from which the pediatric respiratory failure score was developed) and peak inspiratory pressure in the control subjects in the randomized controlled trial were quite different (47 ± 15 vs. 34 ± 6 cm H2O), as were the levels of PaCO2 (56 ± 17 vs. 66 ± 20 mm Hg). In addition, this trial occurred at the time of rising use of high-frequency ventilation (allowed in the control group), inhaled nitric oxide, prone positioning, and surfactant, and while another clinical trial with liquid ventilation was ongoing.28–31 Because of the difficulties observed with this and other randomized trials of therapies in respiratory failure, especially with regards to consensus agreement on entry criteria, patient management techniques, and end points, enthusiasm for another randomized controlled trial of ECLS in pediatric respiratory failure has been dim. Despite the absence of any randomized controlled trial, ECLS continues to be used successfully in an ever-expanding population of children.
Perhaps the most successful randomized trial of ECLS is that recently reported from the UK regarding adult respiratory failure: CESAR (Conventional versus ECMO Support in Adult Respiratory Failure).32 This trial involved adults who were randomized to conventional care or transfered to one adult ECMO center (Glenfield Hospital, Leicester, England) if appropriate inclusion criteria (based on a Murray score >3 or refractory hypercapnia with pH < 7.20) were met. Of 180 patients entered into the CESAR trial, equally divided between conventional and ECMO arms, survival at 6 months without disability was significantly higher in the ECMO arm than in the conventional arm (63% vs. 47%, p = 0.03); the estimated number to treat to achieve an additional life saved was six. The Data Safety and Monitoring Board stopped the trial for efficacy reasons after 180 patients had been entered. An economic analysis concluded that ECLS patients met “acceptable” cost-adjusted quality per life-year costs of £19,252, comparable to treatment of other conditions such as breast cancer.33
Despite the impressive results, the CESAR trial continues to generate controversy, especially regarding the fact that seventeen of the ninety patients randomized to the ECLS arm did not receive ECLS. These seventeen patients were treated at the ECLS center and fourteen survived. In the minds of many clinicians, the finding that patients with severe respiratory failure had improved survival in the ECLS center, whether or not they progressed to the need for ECLS, validated the fact that clinicians in such centers provide optimal care for these patients—and that having ECLS as a tool in the algorithm of care adds a survival benefit.34–36 To others, this fact makes the trial results “invalid” because if the seventeen patients who did not receive ECLS are removed from the analysis, the survival benefit for ECLS is not significantly different from conventional care.
Another criticism of the CESAR trial is that patients managed in the “conventional” sites did not receive a specific algorithm of care, although use of low-tidal volume, pressure-limited ventilation was advocated. To many, the lack of mandated algorithmic care represents the “true” manner in which clinicians in non-ECLS sites provide care and makes the study even closer to what happens in “real life.” To others, the lack of mandated algorithmic care only means that perhaps overall care in the ECLS center was “better,” and the use of ECLS had nothing to do with the observed improved outcome. Also of interest (but without further analysis or explanation) is that patients in the ECLS center received corticosteroids more often than other patients. In summary, the CESAR trial provides important, current-era data and shows a positive benefit for use of ECLS in adult respiratory failure, but also raises questions for continued research and discussion.
On the heels of the publication of the CESAR trial came the H1N1 influenza epidemic of 2009–2010. Early reports of survival in patients with severe respiratory or multiple organ failure secondary to H1N1 from Australia and sites where the epidemic hit before moving west to North America revived interest among many clinicians for use of ECLS in adults. The finding that many patients succumbing to H1N1 were previously healthy young adults may also have impacted the willingness of clinicians to try something outside conventional mechanical ventilation.37–39 Because there are only a few centers in the United States that provide adult ECMO, many ECMO centers were overwhelmed with requests for patient transfer. An H1N1 website developed by the ELSO organization and a U.S. “bed board” map that listed open centers with contact numbers were quickly established to track patients and provide clinicians with needed expertise and assistance. To date, 263 patients have been entered into the ELSO H1N1 database with 63% survival. Two-thirds of patients are older than the age of 18 years.40
The use of extracorporeal techniques today continues to rise in every patient age group. New equipment, better understanding of pathophysiology, improved patient management, and increased interest in ECLS in a wider variety of patients makes it an exciting time in this field.
Criteria for Extracorporeal Life Support
The ability to determine selective criteria for respiratory failure that will separate those patients who will survive with conventional care from those who will not, and thus are most in need of alternative techniques such as ECLS, is a pursuit somewhat similar to the Holy Grail. Despite the creation of multiple severity indices and subsequent evaluation of their efficacy in determining risk of death or morbidity, none have proven sustainable over time or universally correct in predicting outcome.41–46 Nonetheless, the most commonly used criteria for determination of respiratory failure and candidacy for ECLS are the following:
Alveolar-to-arterial oxygen difference (AaDO2), which is calculated using the alveolar gas equation:
- where PB represents barometric pressure (760 mm Hg at sea level), PH2O represents pressure of water vapor (47 mm Hg), and RQ is assumed to be 1. AaDO2 has been historically used in neonatal respiratory failure. An AaDO2 of greater than 610 over 8 hours correlated to 80% mortality in neonatal respiratory failure in historical controls. Among pediatric patients, an AaDO2 of greater than 470 was noted by Timmons to be 81% predictive of death, based on data published in 1991.
Oxygenation index (OI), which is calculated as follows:
- An OI greater than 40 predicted mortality of greater than 80% (historically). An OI of 25 to 40 predicted mortality of 50% to 80% (historically). An OI greater than 40 or remaining greater than 25 over several hours continues to be associated with high mortality in neonates, pediatric patients, and adults (especially in those following lung transplantation). In pediatric respiratory failure, high OI even at seemingly short duration of mechanical ventilation of less than 24 hours, is associated with high mortality, as shown in Figure 20-2.46
Compliance (C), calculated as C = Δvolume/Δpressure, or C = tidal volume/(peak inspiratory pressure* PEEP), although it is preferable to use plateau pressure rather than peak inspiratory pressure. Compliance values of less than 0.5 mL/cm H2O have been used in the selection of adult patients for ECLS.
Intrapulmonary shunt greater than 30% to 50% on FIO2 greater than 0.6. Shunt has been predominantly used as a selection criterion in adult ECLS.
Murray score greater than 3. The score is based on PaO2/FIO2, PEEP, compliance, and number of quadrants exhibiting disease on chest radiograph. The score ranges from 0 to 4, is used in adult ECLS, and was an entry criterion in the CESAR trial.
Ventilatory failure. Hypercarbia with persistent pH less than 7 on high ventilator support, such as peak inspiratory pressure greater than 40 cm H2O.
PaO2/FIO2. Calculation is illustrated by the following example: for PaO2 50 torr, and FIO2 100% (1.0), PaO2/FIO2 is 50/1, or 50. PaO2/FIO2 values of 50 to 100 torr have been described in adult ECLS.
Figure 20-2
Oxygenation index and mortality. The presence of a high oxygenation index at any time during mechanical ventilation was associated with increased risk of death without extubation. (Adapted, with permission, from Trachsel et al.46)
Criteria for Cardiopulmonary Failure
If it is difficult to define criteria for respiratory failure, it is equally so when ECLS is considered for cardiac or multiple organ dysfunction. Although no specific criteria have been identified and universally accepted, the following are suggestions from the literature and clinical experts:47–50
Plasma lactate persistently greater than 5 mM/L.
Mixed venous oxygen saturation (
O2) less than 55% at an estimated cardiac index of at least 2 L/min.
Severe ventricular dysfunction.
Intractable arrhythmia with hemodynamic compromise.
Cardiac arrest.
Inotrope score greater than 50 for 1 hour or greater than 45 for 8 hours,50,51 calculated as
- Dopamine (mcg/kg/min) + Dobutamine (mcg/kg/min) + 100 × Epinephrine (mcg/kg/min).
- A modified inotrope/vasoactive score, calculated as Dopamine (μg/kg/min) + Dobutamine (μg/kg/min) + 100 × Epinephrine (μg/kg/min) + 100 × Norepinephrine (μg/kg/min) + Milrinone (μg/kg/min) × 10 (15 is also used by some clinicians) + 10,000 × vasopressin dose (μg/kg/min).
- Dopamine (mcg/kg/min) + Dobutamine (mcg/kg/min) + 100 × Epinephrine (mcg/kg/min).
Failure to wean from cardiopulmonary bypass.
Venoarterial Cannulation Modes
Venoarterial ECLS has been the predominant mode of support in infants and children for many years secondary to lack of adequately sized venous vessels to obtain the amount of blood flow needed to support the patient. Figure 20-3 is an example of a typical venoarterial ECLS circuit with cervical cannulation, a roller head pump, and silicone membrane lung. Figure 20-4 depicts more current equipment with centrifugal pump and hollow-fiber oxygenator. With the advent of double-lumen, single cannulas, and improvements in flow characteristics of available cannulas, venovenous support is now becoming more popular and feasible even among neonates.51,52 Gone are the days when modifications of thoracostomy or endotracheal tubes provided the “cannulas” for ECLS support; wire-reinforced, thin-walled cannulas now permit excellent flows at smaller internal and external diameters.53 Venoarterial support, however, remains a mainstay of support in patients with combined cardiopulmonary dysfunction or in those with primary cardiac failure. Figures 20-5 and 20-6 show the changes in mode of cannulation by category and diagnostic group.
Figure 20-3
Venoarterial ECLS circuit with roller-head pump and silicone membrane oxygenator. Note venous saturation device on drainage limb; bladder reservoir device is before pump head. Silicone membrane oxygenator has now been almost totally replaced by new hollow-fiber devices. Heat exchanger device rewarms or cools blood before its return to patient.
Figure 20-5
Cannulation mode for patients with respiratory failure (all ages). VA, venoarterial; VA+V, additional venous cannula added; VA-VV, venoarterial cannulation changed to venovenous during ECLS course; VV, venovenous; VVDL, venovenous double lumen; VV-VA, venovenous cannulation changed to venoarterial during ECLS course.
Figure 20-6
Cannulation mode by age. Note increase in venovenous support over past several years. VA, venoarterial; VA+V, additional venous cannula added; VA-VV, venoarterial cannulation changed to venovenous during ECLS course; VV, venovenous; VVDL, venovenous double lumen; VV-VA, venovenous cannulation changed to venoarterial during ECLS course.
In neonates, use of the internal jugular vein and right common carotid artery is the predominant venoarterial cannulation technique. Figure 20-7 is an example of cannulation through the internal jugular vein and carotid artery for venoarterial extracorporeal support with monitoring parameters that can be used for patient management. While centers may vary somewhat in cervical cannulation technique, most use an open approach to vessel access.54 Repair of the carotid artery at decannulation is controversial, practiced routinely in some centers and avoided in others.55–58
Figure 20-7
Venoarterial cannulation. Boxes represent calculations and parameters that can be measured by monitoring site. ACT, activated clotting time; BP, arterial blood pressure; CO, cardiac output (estimated); DO2, oxygen delivery; P, pressure; PAP, pulmonary artery pressure (only in patients with a pulmonary artery catheter in place); PEEP, positive end-expiratory pressure; PPLAT, plateau pressure; PVR, pulmonary vascular resistance; SaO2, arterial oxygen saturation; Sat, saturation of blood; , venous oxygen saturation; SVR, systemic vascular resistance; V, volume;
, carbon dioxide;
oxygen consumption. (Courtesy of Robert Bartlett, MD, with permission.)
Follow-up studies of repaired vessels has noted patency in 80% to 100% of repaired vessels, but stenosis in repaired vessels also has been noted in several studies, as has the development of aneurysms or pseudoaneurysms of the vessel with need for emergent surgical intervention. In one follow-up report of neurodevelopmental outcome from pediatric patients receiving support for cardiac dysfunction, use of the carotid artery for vascular access was not associated with neurologic dysfunction. Similarly, small comparative studies of neonates, with or without carotid repair at decannulation, did not find neurodevelopmental changes at follow-up between groups and noted good collateral flow in patients where the carotid had been ligated. Despite the lack of evidence in favor of repair of the carotid artery, there is increasing interest, especially in older patients, in avoiding the carotid artery for cannulation because the potential risk for stroke seems higher (anecdotally) despite no proven evidence of this concern. Only long-term outcome studies, up to the ages when stroke becomes more common, will be able to definitely answer whether carotid artery ligation or repair following ECLS is an associated risk factor.
Internal jugular cannulation can be performed with a semi-Seldinger technique or via direct venotomy and insertion of the cannula. Some centers also insert a small retrograde venous drainage cannula to the level of the jugular bulb to obtain more venous drainage from the cerebral circuit and/or to monitor cerebral venous saturation as an indication of adequacy of perfusion to the brain and oxygen delivery and extraction (this is presumably even more important in venovenous support).59,60 Some clinicians report having been able to increase venous drainage by up to 30% in neonates with this technique, although others have noted clotting difficulties in the retrograde cannula and have abandoned this technique. The effects of ligation of the internal jugular vein on cerebral venous drainage and risk for intracranial hemorrhage from venous stasis or hypertension has not been completely defined. Repair of the vein at decannulation is not practiced routinely, although patency has been described. For cardiac patients in whom future surgeries may involve use of the superior venous system, such as single ventricle patients, judicious use of the internal jugular vein for ECLS and consideration for repair if cannulation occurs should be discussed.
ECLS can also be provided through direct insertion of large-bore cannulas into the right atrium and into the aortic arch. This method of cannulation allows the largest volume of blood to be obtained for support. This technique is used predominantly in patients transitioned to ECLS directly from cardiopulmonary bypass or following acute deterioration (including arrest) in the early postoperative period when the sternum can be easily reopened.61,62 It has also recently been identified as a potential factor in successful support of patients with septic shock and multiple organ failure, who may require high levels of flow to obtain adequate oxygen delivery. In the most cited report, children in septic shock who received central cannulation were unexpectedly found to have improved survival as compared to those in whom other cannulation strategies were used (73% vs. 38% survival, p = 0.05, n = 11).63 These patients required sternotomy for cannula insertion after peripheral cannulation proved inadequate for support or vascular access could not be obtained. The major differentiating feature between the groups was the higher flow rates obtained with the central cannulation mode. The authors speculated that this improved flow provided better oxygen delivery and resolution of organ failure with resultant improved survival. A follow-up report of twenty-three children with septic shock supported with ECLS over a 9-year period using central cannulation noted 74% survival to discharge.64 Although central cannulation in patients without a prior sternotomy is not routine in most centers, the aforementioned reports have led it to be considered in non–cardiac-surgery patients and we have used it successfully in septic patients in whom peripheral cannulation was ineffective. Bleeding from the mediastinal site continues to be a major complication.
In children older than the age of 2 years or roughly weighing more than 15 kg, femoral vessels may be adequate for venoarterial ECLS. Concern with use of the carotid artery in older patients has already been discussed. Thus, in larger patients (especially adults), femoral cannulation is often preferred. Figure 20-8 is a typical example of femoral/arterial cannulation. Venous cannulation can be performed percutaneously or with open venotomy.65–67 Optimally, a long, large-bore cannula is passed up the femoral vein to the junction of the inferior vena cava-right atrium. Figure 20-9 depicts a typical femoral venoarterial cannulation. It should be noted that as the flow characteristics of a cannula are dependent on internal diameter and length, longer cannulas will have higher resistance to flow; however, achieving access near the right atrium allows for the largest pool of venous blood to be obtained, and, thus, short femoral cannulas (while having less resistance) will frequently not result in adequate venous drainage for ECLS support, especially in small patients. Femoral arterial access can be performed by percutaneous or open cannulation. Typically, a short (18 to 25 cm) cannula is used for ECLS return. In access via the femoral vessels, integrity of distal perfusion or venous drainage of the limb is at risk. Measuring vessel size with bedside ultrasound and picking a cannula with an internal diameter slightly less than the vessel can help allow flow around the cannula and prevent blood flow inadequacies to the distal limb.
Figure 20-8
Modified femoral venoarterial ECMO: For patients usually >15 kg, adequate support can be obtained from femoral venous and femoral arterial cannulation. In this depiction, the patient also has sheaths for cardiac catheterization in the right groin. (Courtesy of Heidi Dalton MD, with permission.)
Figure 20-9
Femoral venoarterial cannulation. Long femoral drainage cannula to right atrium and short arterial return cannula in femoral artery. Boxes represent calculations and parameters that can be measured by monitoring site. ACT, activated clotting time; BP, arterial blood pressure; CO, cardiac output (estimated); DO2, oxygen delivery; P, pressure; PAP, pulmonary artery pressure (only in patients with a pulmonary artery catheter in place); PEEP, positive end-expiratory pressure; PPLAT, plateau pressure; PVR, pulmonary vascular resistance; SaO2, arterial oxygen saturation; Sat, saturation of blood; , venous oxygen saturation; SVR, systemic vascular resistance; V, volume;
, carbon dioxide;
, oxygen consumption. (Courtesy of Robert Bartlett, MD, with permission.)
Placement of small drainage cannulas (for venous engorgement) or distal perfusion cannulas (for distal arterial ischemia) have both been described and used efficiently to prevent compartment syndrome or limb loss.68 Despite these maneuvers, however, limb injury to the extent of amputation has been described several times following femoral access. Thus, close neurovascular monitoring is required.
As venous flow obtained from the femoral route is usually less than that obtained from the internal jugular or central venous sites, the amount of support provided in this mode of venoarterial ECLS is often less than can be obtained with other cannulation techniques. This results in a lower arterial oxygen saturation (SaO2) secondary to increased mixing of desaturated blood from the patient (assuming the patient has severe respiratory failure and impaired gas exchange) than with other forms of venoarterial cannulation. Oxygen saturation often will be 80% instead of 90% to 100% and will mimic that seen with venovenous support. Another important factor with femoral venoarterial support is that the oxygenated arterial return from the ECLS circuit runs retrograde up the aorta to flow coming out of the native heart. How far up the aorta the oxygenated ECLS blood extends is a product of the amount of bypass performed and the amount being ejected from the left ventricle. One concern with femoral venoarterial bypass is that with severe respiratory failure, blood from the left ventricle will be about the same saturation as that in the right atrium, and this relatively desaturated blood will be what is perfusing the upper body, especially the head and heart. This can result in a “blue upper body and red lower body” phenomenon. Whether this is harmful depends on the extent of desaturation and patient-specific factors. For a patient with evidence of myocardial ischemia, having desaturated blood flowing into the coronary vessels may not be optimal. Similarly, if the patient is exhibiting signs of inadequate cerebral oxygenation (by clinical exam or near-infrared spectroscopy monitoring or some other parameter), then this may be an indication that oxygenation is inadequate. If upper-body oxygen delivery is deemed to be significantly impaired, one technique is to place a venous cannula into the right internal jugular vein or in the femoral vein and direct some of the oxygenated ECLS return into this cannula by means of a Y connector on the return limb of the circuit. Figure 20-10 illustrates this method of hybrid femoral venoarterial cannulation with an additional venous cannula providing some oxygenated return. Although the figure depicts two cannulas in one femoral vein, this approach is not common; most clinicians either will place the additional venous cannula in the right internal jugular vein or in the opposite femoral vein. This increases the venous saturation of blood in the right atrium and thus the saturation of blood being ejected out the left ventricle. Careful monitoring of flow in both the venous cannula and the femoral arterial cannula should be performed to prevent one cannula from receiving inadequate flow (as blood will always take the path of least resistance) and clotting. Manipulation of flows to multiple cannulas “Y-ed” into the circuit can be done with a simple screw clamp or other devices. The need for increased venous drainage in femoral venoarterial ECLS can also be achieved by adding another venous cannula (usually in the right internal jugular) and “Y-ing” it into the venous drainage side of the circuit.
Figure 20-10
Hybrid femoral venoarterial cannulation with additional femoral cannula for additional oxygenation to venous circulation. Although the depiction shows additional cannula in same site as venous drainage cannula, it is more common to place additional cannula in the opposite femoral vein or internal jugular vein. Boxes represent calculations and parameters that can be measured by monitoring site. ACT, activated clotting time; BP, arterial blood pressure; CO, cardiac output (estimated); DO2, oxygen delivery; P, pressure; PAP, pulmonary artery pressure (only in patients with a pulmonary artery catheter in place); PEEP, positive end-expiratory pressure; PPLAT, plateau pressure; PVR, pulmonary vascular resistance; SaO2, arterial oxygen saturation; Sat, saturation of blood; , venous oxygen saturation; SVR, systemic vascular resistance; V, volume;
, carbon dioxide;
, oxygen consumption. (Courtesy of Robert Bartlett, MD, with permission.)
Although other vessels, such as the axillary and subclavian, have been used as access for venoarterial ECLS, reports of their use are infrequent. Improved cannula designs with better flow rates and characteristics, may allow improved access to other vessels, even to the point of using umbilical vessels for premature infant support, in the future. Careful attention to neurovascular monitoring is paramount when vessels, especially outside the norm, are cannulated.69
Physiology of Venoarterial Extracorporeal Life Support
Venoarterial ECLS allows for drainage from the right side of the heart, thus reducing blood flow through the native cardiopulmonary circuit. In patients with impaired pulmonary gas exchange, diversion of venous blood into the ECLS circuit, where it undergoes oxygenation and removal of carbon dioxide, and direct return of oxygenated blood into the arterial system allows for increased systemic oxygenation. The more blood diverted into the ECLS circuit, the more bypass is performed and the higher the systemic oxygen delivery provided by ECLS. Increasing ECLS flow will decrease the proportion of blood flowing through the diseased lungs, which is ejected out the left ventricle to mix with the oxygenated return from the ECLS circuit. Thus, at higher ECLS flows, systemic oxygen delivery and SaO2 will increase. This has led to the adage, “If the O’s are low, turn up the flow.” Similarly, at a constant ECLS flow without manipulations in oxygenation of blood within the membrane oxygenator, a simple herald of improving native respiratory function is an increase in SaO2, as the patient’s lungs become more efficient at gas exchange and the arterial oxygen content (CaO2) of ejected left-ventricular blood increases. Just as in normal care of a critically ill patient, understanding the role of CaO2 and oxygen delivery in ECLS is important. As a reminder, CaO2 is calculated as: (hemoglobin g/dL × % SaO2 × 1.36 mL/g) + (0.003 mL/dL × PaO2) × 10. Thus, the major contributors to CaO2 are hemoglobin concentration and SaO2. PaO2 is of little impact on CaO2 unless severe anemia exists. Figure 20-7 shows a typical venoarterial circuit with parameters that can be followed or calculated.
To determine the overall oxygen delivered to the patient during ECLS, both the CaO2 and flow must be considered. The following example illustrates the principles involved:
Assume that perfusate blood is 100% saturated with a PaO2 of 500 torr, as might be seen with 100% FIO2 applied to the membrane lung. If native respiratory failure is so severe that no oxygenation occurs in the pulmonary circuit, blood returning from the native lungs and being ejected out the left ventricle will have the same saturation as right-atrial venous blood. With normal oxygen delivery and extraction, right-atrial venous saturation is 75% and the partial pressure of oxygen (PO2
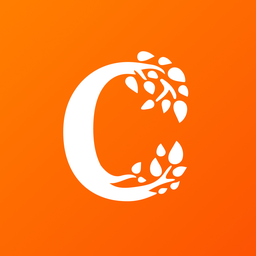
Full access? Get Clinical Tree
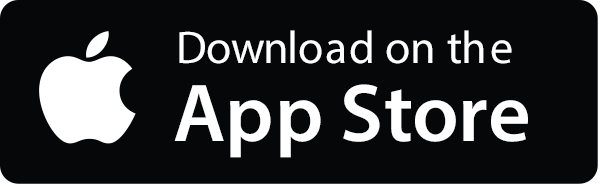
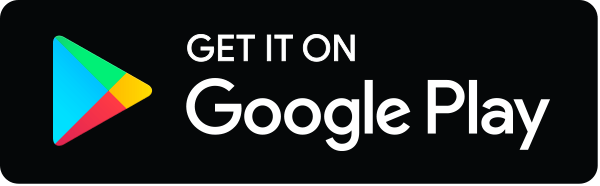
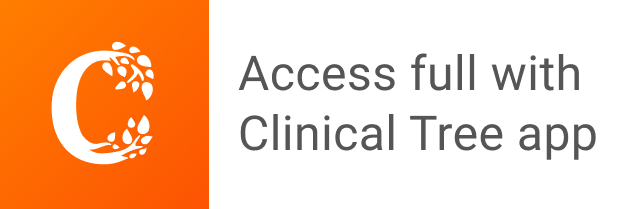