The use of evoked electrophysiological responses during surgery on the nervous system has become a common tool in the operating room. It is used to map the location of structures and to monitor the functional status of neural pathways to enhance intraoperative decision making and improve outcome. These techniques can be used during altered states of consciousness such as during anesthesia or coma. A wide variety of techniques are available and many have been in use since the 1970s.
Basics of evoked potentials
Different than the electroencephalogram (EEG), which records the spontaneous electrical activity of the brain’s cerebral cortex, evoked potentials record the electrical potentials produced after stimulation of specific sensory or motor tracts. The evoked response is recorded as a plot of voltage versus time ( Fig. 6.1 ). It usually has an initial artifact from stimulation of the nerve pathway followed by a series of peaks and valleys. The peaks arise from specific neural generators, often more than one neural structure. The amplitude (peak to adjacent trough) and the latency (time from the stimulation to the peak) are recorded. Peaks are usually named by convention (I through V, P a , P b , or by polarity and latency: P (positive) or N (negative) followed by the latency in milliseconds (msec) (eg, N 20 )).

When the response is large in comparison with background noise, one single evoked response may be sufficient (eg, muscle responses). However, for most sensory responses, the evoked response is very small (1–2 microvolts) compared to other electrical signals (eg, EEG, 10–1000 microvolts). As such signal averaging of many responses is used because the evoked response always occurs at a set time after stimulation, while the background activity acts as a random signal and averages out to zero. Some small responses may need several hundred to thousand signal averages requiring time for the recording.
Evoked responses are used for mapping and monitoring. Mapping involves stimulation and recording to identify the specific location of tracts or stimulation of an unknown structure to determine its function. Monitoring involves repeated stimulation of a neural tract at risk during a procedure to identify impending neural compromise by changes in amplitude or latency. Changes may be due to technical recording problems, alterations in anesthesia management, unfavorable physiological or positioning conditions as well as problems caused by the surgery or procedure.
As a general principle, an amplitude reduction of 50% or latency increase of 10% of a sensory evoked potential is considered significant and raises concern, although smaller changes may indicate impending compromise. For example, ischemia generally produces a loss of response, particularly if synaptic components are involved. Since the tolerance to ischemia (eg, time to irreversible injury) is related directly to the blood flow and metabolic demand, the monitoring may prompt actions to improve blood flow or to improve the tolerance to ischemia in order to reduce the chance of an injury. Fortunately the evoked response is altered at a level of blood flow well above the level that produces irreversible injury.
Somatosensory evoked potentials
The most commonly monitored sensory evoked potential is the somatosensory evoked potential (SSEP). , The SSEP is produced by stimulation of a peripheral sensory nerve with the response measured along the sensory pathway. The large, mixed motor and sensory nerves (and their component spinal roots) that are usually used are median (C6–T1), ulnar (C8–T1), and posterior tibial (L4–S2). Stimulation activates predominantly the large-diameter, fast-conducting Ia muscle afferent and group II cutaneous nerve fibers, which produce the recorded sensory response. Activation of motor fibers in the nerve results in a motor response seen as muscle contraction.
It is currently thought that the neural activity follows the pathway of proprioception and vibration that ascends the spinal cord in the ipsilateral dorsal column. It makes its first synapse near the nucleus cuneatus and nucleus gracilis and then decussates near the cervico-medullary junction, ascending via the contralateral medial lemniscus. A second synapse occurs in the ventro-postero-lateral nucleus of the thalamus, from which it projects to the sensory cortex contralateral to the side of stimulation. For the upper extremity, the evoked responses can be measured from electrodes placed over the antecubital fossa, supraclavicular fossa (brachial plexus), cervical spine, and cortex ( Fig. 6.2 ); for the lower extremity, they can be recorded over the popliteal fossa, along the spinal cord (eg, epidural electrodes), and at cervical and cortical locations. Recordings are usually conducted at multiple recording sites to verify that the nervous system is stimulated and to identify the anatomic location of neural compromise if the response is lost.
The cortical response is most frequently recorded over the primary somatosensory cortex of the sensory area of the nerve stimulated. The major cortical peaks are probably the result of the thalamocortical projections to the primary sensory cortex. Responses recorded posteriorly over the cervical spine (subcortical response) probably represent responses of the tracts in the spinal cord or brainstem.
Mapping Using Somatosensory Evoked Potentials
The SSEP can be used for mapping in the spinal cord and the somatosensory cortex. For example, if the surgeon wishes to make a midline posterior myelotomy for entry into the spinal cord to operate on a spinal cord tumor, the midline can be identified by placing a series of electrodes across the posterior spinal cord. The midline lies between the maximal response recorded from individual stimulation of the left and the right peripheral nerves.
The SSEP can also be used to identify the location of the sensory strip on the cerebral cortex. Localization is accomplished by recording of the cortical component (N 20 ) of the median nerve SSEP with use of bipolar recording strips placed on the cortex. The central gyrus separating the motor and sensory strips is identified from a phase reversal (initial wave changes from positive to negative) of the response.
Cortical Monitoring Using Somatosensory Evoked Potentials
The cortical SSEP has been used to detect ischemia in cortical tissue by amplitude reduction. Although the clinical examination becomes abnormal at a cortical blood flow of about 25 mL/min/100 g, the SSEP becomes abnormal at about 20 mL/min/100 g and is lost at 15 and 18 mL/min/100 g. Subcortical regions, brainstem, spinal cord, and nerve appear to be less sensitive to hypoperfusion. Hence, the SSEP is used to monitor procedures such as carotid endarterectomy (CEA) where changes have been used as an indication for shunt placement and to predict postoperative morbidity. In some respects, use of the EEG and SSEP in CEA are complimentary because the SSEP is able to detect ischemia in deep cortical structures, and the EEG assesses a wider area of surface cortex.
Similarly, the SSEP is employed during intracranial vascular procedures to determine the adequacy of collateral blood flow, tolerance to temporary vessel occlusion, or the adequacy of systolic blood pressure. Because the SSEP from the upper extremity is generated in the cerebral cortex supplied by the middle cerebral artery, it can be utilized during surgery for aneurysms of the internal carotid and middle cerebral arteries. Similarly, the SSEP from the lower extremity can be used to evaluate ischemia during vascular procedures on the anterior cerebral artery. Monitoring of ischemia in these arteries may require both upper and lower SSEPs, depending on the vascular perforators (eg, lenticulate striate perforators supplying the internal capsule) and their impact on the subcortical pathways (eg, the descending motor tracts requiring motor evoked potentials as below).
Monitoring during temporary clipping in aneurysm surgery has shown that a very prompt loss of cortical SSEP response (less than 1 minute after clipping) is associated with a permanent neurologic deficit. However, a delayed loss with prompt recovery after release of the clip is associated with collateral circulation and a markedly reduced incidence of neural morbidity. Symon and colleagues have suggested that when the N 20 of the median nerve SSEP disappears slowly (over 4 minutes), 10 additional minutes of occlusion can be tolerated safely. A correlation between outcome and monitoring during anterior circulation aneurysms has been observed.
In addition, monitoring can be used to identify ischemia from vasospasm or when a combination of factors produces unexpected ischemia (eg, retractor pressure, hypotension, temporary clipping, and hyperventilation). SSEP monitoring during neuroradiological procedures like occlusion of vessels or during streptokinase dissolution of blood clots is equally effective.
Somatosensory Evoked Potential Monitoring in Spinal Surgery
The SSEP can be used during brainstem or spinal procedures. Perhaps the most common application has been to reduce the risks of spinal cord or axial surgery where it can identify mechanical or ischemic insults ( Fig. 6.3 ). Monitoring is estimated to reduce the morbidity in spinal surgery by 50– 80%.
The basis of monitoring spinal surgery in humans is founded in studies in animals where compromise of the spinal cord results in SSEP latency and amplitude changes simultaneous to loss of clinical motor function. Because current spinal instrumentation techniques present multiple potential insults, a nearly continuous monitoring technique (eg, SSEP) is an important advantage for identifying a specific insult. In addition, the SSEP may identify physiologic insults (eg, hypotension) or positioning problems. The once frequently used wake-up test is utilized less since the introduction of SSEP monitoring and is now often reserved for assessment of motor function when motor evoked potentials (MEPs) are not recordable or to confirm motor function when evoked responses deteriorate.
Studies in humans also indicate that the SSEP is predictive of neural outcome. , The utility of the SSEP in spinal surgery was shown by the Scoliosis Research Society and European Spinal Deformities Society in 1995. In 51,263 spinal deformity operations (scoliosis, kyphosis, fractures, and spondylolisthesis) the overall injury incidence was 0.55%; well below the 0.7–4% historical average without monitoring. This and other studies led the Scoliosis Research Society to develop a position statement that made monitoring a virtual standard of care.
Based on these results, Nuwer estimated the economic impact of SSEP monitoring. Approximately 200 cases at a cost of $120,000 (1995 dollars) were required to prevent one major, persistent neurologic deficit, which is small compared with the cost of lifelong medical care.
However, the correlation of SSEP and neural injury is not exact; the incidence of a major motor injury without SSEP warning was 0.063% (about 1 in 1500 procedures). This highlights the anatomic difference of the SSEP and motor pathways in the spinal cord. Therefore, the ability of the SSEP to predict most motor deficits probably results from insults that affect the entire cross-section of the spinal cord. More recent evidence-based reviews reinforce the use of monitoring in spine surgery. ,
Recording Somatosensory Evoked Potentials from the Spinal Column
The spine has also been monitored using stimulation and recording from epidural or electrodes in the bony spine in an attempt to monitor motor tracts. Problems with these techniques include lack of laterality and that the actual neural tracts monitored are not known. The MEP (see below) has generally replaced spinal stimulation techniques since they may be primarily assessing sensory tracts.
Somatosensory evoked potentials
The most commonly monitored sensory evoked potential is the somatosensory evoked potential (SSEP). , The SSEP is produced by stimulation of a peripheral sensory nerve with the response measured along the sensory pathway. The large, mixed motor and sensory nerves (and their component spinal roots) that are usually used are median (C6–T1), ulnar (C8–T1), and posterior tibial (L4–S2). Stimulation activates predominantly the large-diameter, fast-conducting Ia muscle afferent and group II cutaneous nerve fibers, which produce the recorded sensory response. Activation of motor fibers in the nerve results in a motor response seen as muscle contraction.
It is currently thought that the neural activity follows the pathway of proprioception and vibration that ascends the spinal cord in the ipsilateral dorsal column. It makes its first synapse near the nucleus cuneatus and nucleus gracilis and then decussates near the cervico-medullary junction, ascending via the contralateral medial lemniscus. A second synapse occurs in the ventro-postero-lateral nucleus of the thalamus, from which it projects to the sensory cortex contralateral to the side of stimulation. For the upper extremity, the evoked responses can be measured from electrodes placed over the antecubital fossa, supraclavicular fossa (brachial plexus), cervical spine, and cortex ( Fig. 6.2 ); for the lower extremity, they can be recorded over the popliteal fossa, along the spinal cord (eg, epidural electrodes), and at cervical and cortical locations. Recordings are usually conducted at multiple recording sites to verify that the nervous system is stimulated and to identify the anatomic location of neural compromise if the response is lost.
The cortical response is most frequently recorded over the primary somatosensory cortex of the sensory area of the nerve stimulated. The major cortical peaks are probably the result of the thalamocortical projections to the primary sensory cortex. Responses recorded posteriorly over the cervical spine (subcortical response) probably represent responses of the tracts in the spinal cord or brainstem.
Mapping Using Somatosensory Evoked Potentials
The SSEP can be used for mapping in the spinal cord and the somatosensory cortex. For example, if the surgeon wishes to make a midline posterior myelotomy for entry into the spinal cord to operate on a spinal cord tumor, the midline can be identified by placing a series of electrodes across the posterior spinal cord. The midline lies between the maximal response recorded from individual stimulation of the left and the right peripheral nerves.
The SSEP can also be used to identify the location of the sensory strip on the cerebral cortex. Localization is accomplished by recording of the cortical component (N 20 ) of the median nerve SSEP with use of bipolar recording strips placed on the cortex. The central gyrus separating the motor and sensory strips is identified from a phase reversal (initial wave changes from positive to negative) of the response.
Cortical Monitoring Using Somatosensory Evoked Potentials
The cortical SSEP has been used to detect ischemia in cortical tissue by amplitude reduction. Although the clinical examination becomes abnormal at a cortical blood flow of about 25 mL/min/100 g, the SSEP becomes abnormal at about 20 mL/min/100 g and is lost at 15 and 18 mL/min/100 g. Subcortical regions, brainstem, spinal cord, and nerve appear to be less sensitive to hypoperfusion. Hence, the SSEP is used to monitor procedures such as carotid endarterectomy (CEA) where changes have been used as an indication for shunt placement and to predict postoperative morbidity. In some respects, use of the EEG and SSEP in CEA are complimentary because the SSEP is able to detect ischemia in deep cortical structures, and the EEG assesses a wider area of surface cortex.
Similarly, the SSEP is employed during intracranial vascular procedures to determine the adequacy of collateral blood flow, tolerance to temporary vessel occlusion, or the adequacy of systolic blood pressure. Because the SSEP from the upper extremity is generated in the cerebral cortex supplied by the middle cerebral artery, it can be utilized during surgery for aneurysms of the internal carotid and middle cerebral arteries. Similarly, the SSEP from the lower extremity can be used to evaluate ischemia during vascular procedures on the anterior cerebral artery. Monitoring of ischemia in these arteries may require both upper and lower SSEPs, depending on the vascular perforators (eg, lenticulate striate perforators supplying the internal capsule) and their impact on the subcortical pathways (eg, the descending motor tracts requiring motor evoked potentials as below).
Monitoring during temporary clipping in aneurysm surgery has shown that a very prompt loss of cortical SSEP response (less than 1 minute after clipping) is associated with a permanent neurologic deficit. However, a delayed loss with prompt recovery after release of the clip is associated with collateral circulation and a markedly reduced incidence of neural morbidity. Symon and colleagues have suggested that when the N 20 of the median nerve SSEP disappears slowly (over 4 minutes), 10 additional minutes of occlusion can be tolerated safely. A correlation between outcome and monitoring during anterior circulation aneurysms has been observed.
In addition, monitoring can be used to identify ischemia from vasospasm or when a combination of factors produces unexpected ischemia (eg, retractor pressure, hypotension, temporary clipping, and hyperventilation). SSEP monitoring during neuroradiological procedures like occlusion of vessels or during streptokinase dissolution of blood clots is equally effective.
Somatosensory Evoked Potential Monitoring in Spinal Surgery
The SSEP can be used during brainstem or spinal procedures. Perhaps the most common application has been to reduce the risks of spinal cord or axial surgery where it can identify mechanical or ischemic insults ( Fig. 6.3 ). Monitoring is estimated to reduce the morbidity in spinal surgery by 50– 80%.
The basis of monitoring spinal surgery in humans is founded in studies in animals where compromise of the spinal cord results in SSEP latency and amplitude changes simultaneous to loss of clinical motor function. Because current spinal instrumentation techniques present multiple potential insults, a nearly continuous monitoring technique (eg, SSEP) is an important advantage for identifying a specific insult. In addition, the SSEP may identify physiologic insults (eg, hypotension) or positioning problems. The once frequently used wake-up test is utilized less since the introduction of SSEP monitoring and is now often reserved for assessment of motor function when motor evoked potentials (MEPs) are not recordable or to confirm motor function when evoked responses deteriorate.
Studies in humans also indicate that the SSEP is predictive of neural outcome. , The utility of the SSEP in spinal surgery was shown by the Scoliosis Research Society and European Spinal Deformities Society in 1995. In 51,263 spinal deformity operations (scoliosis, kyphosis, fractures, and spondylolisthesis) the overall injury incidence was 0.55%; well below the 0.7–4% historical average without monitoring. This and other studies led the Scoliosis Research Society to develop a position statement that made monitoring a virtual standard of care.
Based on these results, Nuwer estimated the economic impact of SSEP monitoring. Approximately 200 cases at a cost of $120,000 (1995 dollars) were required to prevent one major, persistent neurologic deficit, which is small compared with the cost of lifelong medical care.
However, the correlation of SSEP and neural injury is not exact; the incidence of a major motor injury without SSEP warning was 0.063% (about 1 in 1500 procedures). This highlights the anatomic difference of the SSEP and motor pathways in the spinal cord. Therefore, the ability of the SSEP to predict most motor deficits probably results from insults that affect the entire cross-section of the spinal cord. More recent evidence-based reviews reinforce the use of monitoring in spine surgery. ,
Recording Somatosensory Evoked Potentials from the Spinal Column
The spine has also been monitored using stimulation and recording from epidural or electrodes in the bony spine in an attempt to monitor motor tracts. Problems with these techniques include lack of laterality and that the actual neural tracts monitored are not known. The MEP (see below) has generally replaced spinal stimulation techniques since they may be primarily assessing sensory tracts.
Auditory brainstem responses
The auditory brainstem response (ABR) is a sensory evoked potential which is produced when sound activates the auditory pathway. The term auditory brainstem response refers to the responses from the brainstem in the first 10 msec after stimulation ( Fig. 6.4 ). They are also referred to as the brainstem auditory evoked response (BAER) and brainstem auditory evoked potential (BAEP). The sound activates the cochlea and the resulting nerve impulse travels via the eighth cranial nerve, the brainstem acoustic nuclei, and lemniscal pathways, eventually to activate the auditory cortex. Five ABR peaks are seen (numbered I–V) with monitoring of I, III, and V. It is postulated that wave I is produced by the extracranial portion of cranial nerve (CN) VIII, wave III by the cochlear nucleus, and wave V by the lateral lemniscus and inferior colliculus in the contralateral pons.
The ABR is used extensively for monitoring during posterior fossa surgery, because of its importance for hearing and the frequent involvement of the cochlear nerve by tumors in this region. , Quite resistant to the effects of anesthesia, a variety of factors alter the ABR. They include sound conduction problems in the external or middle ear, ischemia of the cochlea, traction on cranial nerve VIII, and ischemia or neural damage to the auditory pathways in the brainstem.
Common changes seen intraoperatively with ABR recordings are increases in the latency of wave V and increases in the interpeak latency of I–V with retractor placement in the posterior fossa. These changes, if mild, are reversible and considered part of a routine procedure. Complete loss of wave I can be due to the loss of the cochlear blood supply as a result of vascular obstruction or vasospasm or transection of the nerve by the surgeon. In general, if waves I and V are preserved, hearing is preserved, but if they are both lost, there is little chance of preservation of hearing postoperatively.
ABRs are also used to evaluate general brainstem viability such as decompression of space-occupying defects in the cerebellum, removal of cerebellar vascular malformations, and microvascular decompression for relief of hemifacial spasm or trigeminal neuralgia. The SSEP and motor evoked potentials are typically combined with ABRs to more comprehensively monitor brainstem integrity.
Visual evoked potentials
Visual evoked potentials (VEPs) are produced in response to light stimulation of the eyes (see Fig. 6.1 ). The most commonly recorded responses appear to be generated bilaterally in the visual (occipital) cortex. Under anesthesia, flash stimulation through closed eyelids or stimulators mounted on scleral caps are used. The response of the retina (electroretinogram [ERG]) can also be measured. They are considered less useful in surgical monitoring because flash stimulation does not appear to measure the pathways of useful clinical vision, the typical large, bulky “goggles” pose technical problems, anesthetic sensitivity, and that the bilateral response from either eye obscures some focal changes.
Basic electromyographic monitoring
Recording muscle electrical activity using needle electrode pairs is called electromyography (EMG). Two types of intraoperative EMG monitoring are common: recording spontaneous activity and recording triggered responses subsequent to stimulation of motor nerves or motor pathways. The responses are sufficiently large (0–1.5 millivolts) so averaging is not necessary allowing immediate feedback. Spontaneous activity is observed continuously with stimulation used for specific activities. Direct feedback to the surgeon can be achieved by the playing of these responses through a loudspeaker. EMG monitoring has found wide application in surgery of the posterior fossa, skull base, spine, cauda equina, head and neck, and peripheral nerves.
The EMG response that results from irritation of a nerve is a recording of the generated motor unit potentials of individual axon-muscle fiber groups. Neurotonic discharges, caused by mechanical or metabolic stimuli, are high-frequency intermittent or continuous bursts of motor unit potentials and are a sensitive indicator of nerve irritation ( Fig. 6.5 ). Activity can be bursts lasting less than 200 msec with single or multiple motor unit potentials firing at 30 to 200 Hz, or they can be long trains of activity lasting 1 to 30 seconds or more. Short bursts represent nerve irritation exemplified by relatively synchronous motor unit discharges from a response of multiple axons from nerve irritation. When these are of sufficient amplitude or duration they raise concern. Causes of irritation include nearby mechanical stimulation (eg, dissection, ultrasonic aspiration or drilling), nerve retraction, thermal irritation (eg, heating from irrigation, lasers, drilling, or electrocautery), and chemical or metabolic insults. Prolonged irritation may lead to long trains of continuous, synchronous motor unit discharges and are associated with impending nerve injury (nerve compression, traction, or ischemia of the nerve). Sharp transection of nerves may fail to provoke any discharge.
Direct stimulation of neural tissue can be used for mapping to locate a nerve of interest or to assess its function. The electrical responses recorded from intentional nerve stimulation (to determine whether the neural tract is intact) or MEPs (see later) are called compound muscle action potentials (CMAPs). The nerve is stimulated by bipolar or monopolar electrodes with fine tips. The EMG response that results from single or multiple stimuli of low-intensity current (0.5–5 milliAmperes [mA]) and short duration (0.5–1 msec) will identify cranial nerves or assess damaged peripheral nerves.
Mapping techniques include stimulation to identify motor cranial nerves (CN) near or intertwined in brainstem tumors or identify the location of cranial nerve nuclei on the posterior surface of the brainstem. Locating CN nuclei allows “safe entry” zones to be located for surgery to resect deeper pathology. It has also been used to identify nerve rootlets for resection to minimize childhood spasticity. Finally, mapping is extensively used in surgery on the cauda equina to determine if tissue tethering the spinal cord is functional (particularly to preserve key roots for anal and urethral sphincter control).
The EMG is resistant to the effects of most physiologic variables (temperature, blood pressure) and anesthetics, except for neuromuscular blockade (NMB). Electrically stimulated responses can usually be monitored with NMB with 75% or less suppression of baseline CMAPs. Small-amplitude responses from irritation, especially in damaged or poorly functioning nerves, may be difficult to detect during controlled muscle relaxation such that partial NMB should be used only when necessary.
Cranial Nerve Monitoring
EMG is used to monitor cranial nerves because they are susceptible to intraoperative damage owing to their small size, limited epineurium, and complicated course. The damage occurs from either surgical trauma or ischemia and leads to paresis or paralysis with subsequent disability or deformity and, possibly, chronic pain. Cranial nerves are at risk during excision of tumors of the skull base, brainstem, and cerebellopontine angle. All cranial nerves with a motor component can be monitored using their respectively innervated muscles ( Table 6.1 ).
Cranial Nerve | Monitoring Site or Method * | |
---|---|---|
I | Olfactory | No monitoring technique |
II | Optic | Visual evoked potentials |
III | Oculomotor | Inferior rectus muscle |
IV | Trochlear | Superior oblique muscle |
V | Trigeminal | Masseter muscle and/or temporalis muscle (sensory responses can also be monitored) |
VI | Abducens | Lateral rectus muscle |
VII | Facial | Orbicularis oculi and/or orbicularis oris muscles |
VIII | Auditory | Auditory brainstem responses |
IX | Glossopharyngeal | Stylopharyngeus muscle (posterior soft palate) |
X | Vagus | Vocal folds, cricothyroid muscle |
XI | Spinal accessory | Sternocleidomastoid and/or trapezius muscles |
XII | Hypoglossal | Genioglossus muscle (tongue) |
* Unless otherwise specified, monitoring is performed via electromyographic activity of the muscle(s) listed.
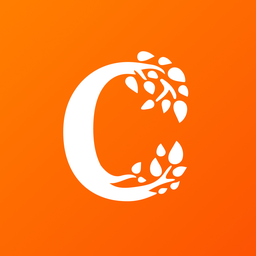
Full access? Get Clinical Tree
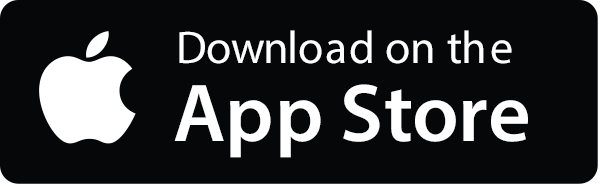
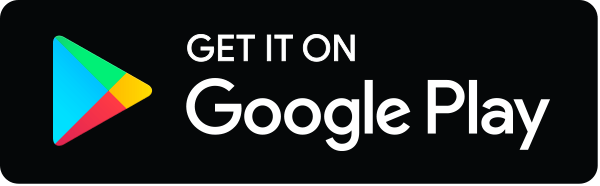