CHAPTER 10 Equipment
Humidifiers
During normal breathing, the nasal mucosa and upper airway serve as a reservoir for condensed water. This water is evaporated during breathing and reduces the heat expended by the body in warming and humidifying inhaled gases. In typical atmospheric conditions, air in the pulmonary periphery contains 44 mg of water per liter at 37° C (100% relative humidity). Breathing ambient air at a temperature of 22° C, water content of 10 mg/dL would require the addition of 34 mg/L of evaporated water. This evaporation results in a loss of energy with the resultant cooling of the respiratory mucosa. The caloric expenditure of humidification consumes approximately five times the energy required to heat the inspired gases; this may amount to 20% of the basal metabolic rate of an infant (Rashad and Benson, 1967). Nasopharyngeal humidification is bypassed with the placement of common airway devices such as tracheal tubes, tracheostomy tubes, and laryngeal masks. Benefits of heating and humidifying anesthetic gases include prevention of intraoperative hypothermia, decreased atelectasis, and improved mucociliary clearance. Consequences of overhumidification include impaired mucociliary clearance related to reduced mucus viscosity, atelectasis, accumulation of secretions, infection, thermal injury, and surfactant inactivation (Schiffmann, 2006). Partial humidification of gas in the anesthesia breathing circuit takes place within the carbon-dioxide absorber, which uses an exothermic reaction that may raise the water vapor content to as much as 29 mg/L. Further humidification is accomplished by reducing the amount of fresh-gas flow, thereby increasing rebreathing of humidified gases and using a heat and moisture exchanger (HME, or “artificial nose”). The HME uses a fine mesh to cause condensation of exhaled water vapor. The HME may increase the resistance to breathing or the dead space for some infants and children, although these changes are usually tolerable. Low fresh-gas flow alone is often inadequate at maintaining humidity and temperature in infants and neonates, therefore active or passive humidification devices should always be incorporated when ventilating this population (Hunter et al., 2005). HMEs increase airway humidification and preserve temperature in anesthetized children at a lower cost than active humidification systems (Bissonnette and Sessler, 1989) and they require 80 minutes to achieve optimal saturation of the membrane, during which time they are less efficient. HMEs come in a variety of sizes, enabling selection based on size of the patient, so that dead space or resistance can be minimized. Specially designed HMEs filter out infectious pathogens and minimize the risk of cross-infection between patients (Wilkes et al., 2000).
Active humidification is the most efficient means by which to heat and humidify inspired gases (Bissonnette and Sessler, 1989). A servocontrolled, shielded heated wire in the fresh gas line helps to prevent cooling and condensation of the water as it passes through the inspiratory limb. The temperature should be regulated by a probe near the patient connection, because overheating of the inspired gases can produce injury to the airway (Klein and Graves, 1974). Active humidifiers may also increase the compression volume of the breathing circuit; thus, compensatory increases in the tidal volume during controlled ventilation may be necessary except in ventilators with compliance compensation (Coté et al., 1983).
Anesthesia breathing systems
Since Philip Ayre’s (1937) landmark article began the modern era of breathing systems for pediatric anesthesia, this has been a topic of controversy. Using Magill’s technique of tracheal intubation for the repair of cleft lip and palate in infants, Ayre noted adverse results. Breathing through a “closed” high-resistance system, these infants often developed “rapid, ‘sighing’ respirations” and “ashy pallor and sweating.” They exhibited a “dark, congested oozing at the site of operation.” Postoperatively, the infants were “in varying degrees of shock: some…for days” (Ayre, 1937). The contribution of hypotension or hypovolemia to this picture remains unknown, because blood pressure was not measured, and blood loss was difficult to quantify by Ayre’s account.
Ayre noted dramatic clinical improvement when he adopted an open T-piece breathing system. The T piece, an extremely simple device, consists of an inspiratory limb, a connection to the patient, and an expiratory limb. It has no unidirectional or overflow valves, and there is no breathing bag. The expiratory limb serves as a reservoir for fresh gas, a means of monitoring the infant’s respirations, and if the distal end is intermittently occluded, a means of providing positive pressure ventilation. If the volume of the expiratory limb is one third of the tidal volume, rebreathing can be virtually eliminated during spontaneous ventilation with a fresh-gas flow that is twice the minute ventilation (Ayre, 1956). Ayre attributed the salutary effect of the T piece to marked reductions in resistance to gas flow and rebreathing.
Nonrebreathing and Partial Rebreathing Systems
Despite its apparent benefits, the T piece is far from ideal. The major flaws are its release of anesthetic gases into the operating room and its inability to provide assisted or controlled ventilation. A series of modifications occurred. Rees (1950) first proposed the addition of a breathing bag to the expiratory limb. Another system, the Magill attachment, which predated Ayre’s publication, introduced fresh gas distal to a breathing bag and an overflow valve near the patient connection. These and other variations were brought together under a single classification scheme proposed by Mapleson (1954), in which each system was distinguished on the basis of the location of its fresh gas inflow and overflow valves relative to the patient connection.
These Mapleson circuits share the benefit of reduced resistance to breathing by virtue of the absence of unidirectional valves and canisters; the elimination of these components results in varying degrees of rebreathing that depend on the fresh-gas flow (Fig. 10-1, A). Rebreathing is not necessarily bad because it serves to conserve heat, humidity, and anesthetic gases. Yet in the absence of a mechanism by which to monitor the accumulation of carbon dioxide, the consequences of hypercarbia and respiratory acidosis probably outweigh these benefits.
Mapleson A System
The Mapleson A system results in no rebreathing during spontaneous ventilation when the fresh-gas flow is more than 75% of the minute ventilation; it requires a larger fresh-gas flow to eliminate rebreathing during controlled ventilation (Fig. 10-1, B) (Waters and Mapleson, 1961; Kain and Nunn, 1967). This design is impractical in the operating room, because the proximal location of the overflow valve makes it cumbersome for scavenging waste gases, difficult to adjust during head-and-neck surgery, and potentially dangerous, because the heavy valve could dislodge a small tracheal tube.
Mapleson D System
The Mapleson D system is characterized by a proximal fresh-gas inflow and a distal overflow valve. It is a modification of the T piece, in which a breathing bag and an overflow valve have been added to the distal expiratory limb. Although it requires slightly more fresh-gas flow to eliminate rebreathing during spontaneous ventilation than the Mapleson A system, it is the most economical during controlled ventilation (Waters and Mapleson, 1961). On balance, considering both spontaneous and controlled ventilation, the Mapleson D requires the lowest fresh-gas flow rates among all Mapleson circuits. This system has become the most widely used of the Mapleson circuits for pediatric anesthesia.
The precise flow dynamics in the Mapleson D system have been a subject of discussion that has resulted in a variety of complex recommendations (Mapleson, 1954; Waters and Mapleson, 1961, Nightingale et al., 1965; Bain and Spoerel, 1973; Rose et al., 1978; Spoerel et al., 1978; Rose and Froese, 1979). To eliminate rebreathing, higher fresh-gas flows are needed during spontaneous ventilation than during controlled ventilation. With spontaneous ventilation, rebreathing is eliminated by provision of fresh-gas flow equal to the mean inspiratory flow rate (Mapleson, 1954; Rose et al., 1978). If an inspiratory/expiratory ratio is 1:1 or 1:2, the mean inspiratory flow rate is 2 to 3 times the minute ventilation. Although Spoerel and others (Bain, 1979) have demonstrated that a normal arterial carbon dioxide tension (Paco2) can be maintained during spontaneous ventilation at fresh-gas flows as low as 100 mL/kg per minute, an increased minute ventilation (and hence more respiratory work) is required to compensate for rebreathed carbon dioxide.
The recommendations for fresh-gas flow during controlled ventilation are complex and varied (Waters and Mapleson, 1961; Nightingale et al., 1965; Bain and Spoerel, 1973). This reflects the importance of several factors that were summarized by Rose and Froese (1979) (Fig. 10-2). When a high fresh-gas flow (greater than 100 mL/kg per minute) is used, the Paco2 is governed by minute ventilation (ventilation limited). At low fresh-gas flow (less than 90 mL/kg per minute), Paco2 is independent of minute ventilation, varying instead as a function of the amount of rebreathing, which is governed by the fresh-gas–flow rate (flow limited).
Additional important factors that govern the magnitude of rebreathing include carbon dioxide production, respiratory rate, and respiratory waveform characteristics (e.g., inspiratory flow, inspiratory and expiratory times, and expiratory pause) (Rose and Froese, 1979). Adjustments to the ventilatory pattern that allow the fresh-gas flow to constitute a larger proportion of the inspired gas (e.g., slow inspiratory time or low inspiratory flow) or that enable exhaled gases to be more completely washed out (e.g., long expiratory pause or slow rate) reduce the amount of rebreathing. With a Mapleson D circuit that has controlled ventilation and low fresh-gas flow (flow limited), an attempt to reduce the Paco2 by increasing the respiratory rate would reduce the expiratory pause and thus promote rebreathing (Fig. 10-3). In this situation, the increased ventilation is offset by increased fraction of inspired oxygen (Fico2), resulting in no net change in Paco2. To wash out the exhaled gas at this higher respiratory rate and take advantage of the increased minute ventilation, the fresh-gas flow must be increased. These fresh-gas flow and ventilatory recommendations are predicated for a normal metabolic rate and hence normal carbon dioxide production (Bain and Spoerel, 1977; Nightingale and Lambert, 1978). Conditions that increase carbon dioxide production (e.g., fever, catabolic state, or malignant hyperthermia) must be met with a proportional increase in fresh-gas flow or ventilation.
Bain Modification of Mapleson D
The Bain modification of the Mapleson D circuit incorporates the fresh-gas supply within the expiratory limb in a coaxial arrangement (Fig. 10-4) (Bain and Spoerel, 1972). This circuit is light and streamlined with only a single hose to the patient. It also provides some countercurrent warming of the inspired gases and effective scavenging of expired gases. Its major disadvantage lies in the inability to directly inspect the integrity of the inspiratory limb. Pethick (1975) described an indirect test of the Bain inspiratory-limb integrity in which the oxygen flush is passed through the circuit for several seconds. If the inspiratory limb is intact, the rapid flow of gas through it exerts a Venturi effect on the expiratory limb, resulting in a slight negative pressure and collapse of the breathing bag. With a leak from the inspiratory limb into the expiratory limb, the pressure in the latter rises, tending to inflate the reservoir bag. The rebreathing characteristics of the Bain circuit are identical to those of any other Mapleson D. The major reasons that proponents have advocated the use of these Bain circuits for pediatric anesthesia are their relatively lower resistance to breathing offered by an open system, the countercurrent warming of gases, and the low profile of a single hose attached to the patient.
The primary sources of resistance in an anesthesia delivery system are the tracheal tube, the valves, and the carbon dioxide absorber. With modern equipment, the tracheal tube represents a major source of resistance in the neonate (Cave and Fletcher, 1968; Brown and Hustead, 1969). Light-weight, large-diameter, modern disc valves exert resistance in two ways. There is a minimum, flow-independent resistance necessary to displace the valve, usually much less than 1 cm H2O (Hunt, 1955). A much higher resistance may be required when the expiratory valve is wet. At high gas flows (more than 30 L/min), the valves also become a source of turbulent resistance proportional to the flow through them. Carbon dioxide canisters are also a source of turbulence. Their resistance is inversely proportional to the length of the path the gas must take through the resistor. Modern absorbers are short and wide to minimize this path of resistance.
Approximately a half century after Ayre introduced the T piece, the extent to which his work applies remains unclear. Perhaps the infants Ayre studied were subjected to the significant resistance imposed by the valves of that era. Although the neonate has a lower proportion of fatigue-resistant fibers in the diaphragm, infants as young as 2 weeks old have been shown to compensate for increases in resistance of 200% without changes in blood gases, at least for relatively short lengths of time (Muller et al., 1979). The benefits of the Mapleson systems must be weighed against their inherent problems on an individual basis. If a practitioner seldom uses Mapleson circuits and is unfamiliar with their characteristics, or if a practitioner has to make substantial alterations to an anesthesia machine to accommodate them, the additional risks of the situation must be considered. Even if a circle system is used for most children, it is important to understand the flow requirements of these circuits, as they continue to be used for resuscitation outside of the operating room and for transporting critically ill patients.
Circle Systems
The circle system is standard equipment on anesthesia machines that have been designed to meet the standards specified in ASTM 1850-00 (2005). When functioning properly, it enables lower fresh-gas flows than the Mapleson D circuit; a circle system conserves heat, humidity, and anesthetic agent. It also minimizes environmental pollution. Compared with the resistance of an endotracheal tube, the additional resistance imposed by the addition of unidirectional valves and a carbon-dioxide canister is trivial. Because the ratio of the patient’s tidal volume to the volume of the inspiratory limb is small in young children, changes in the anesthetic concentration can take some time to reach equilibrium unless higher fresh-gas flows are used. It is important to be vigilant for the manifestations of stuck (resistance) or floating (rebreathing) unidirectional valves, because both have harmful consequences in small children.
Anesthesia machines
Gas and Anesthetic Vapor Delivery
Oxygen
All modern anesthesia machines are designed to ensure continuous oxygen delivery from either pipeline or cylinder supplies and to notify the user if the oxygen-supply pressure fails. Because pressure in the oxygen supply does not guarantee that oxygen is indeed flowing through the pipes, an oxygen analyzer located in the inspiratory limb of the anesthesia circuit is an essential safety monitor for every anesthetic (Dorsch and Dorsch, 2007). Indeed, an inspired oxygen-concentration monitor is considered to be the standard of care for every anesthetic according to the American Society of Anesthesiologists (2005) (Box 10-1).
Box 10-1 Standards for Basic Anesthetic Monitoring Committee of Origin: Standards and Practice Parameters
These standards apply to all anesthesia care although, in emergency circumstances, appropriate life support measures take precedence. These standards may be exceeded at any time based on the judgment of the responsible anesthesiologist. They are intended to encourage quality patient care, but observing them cannot guarantee any specific patient outcome. They are subject to revision from time to time, as warranted by the evolution of technology and practice. They apply to all general anesthetics, regional anesthetics and monitored anesthesia care. This set of standards addresses only the issue of basic anesthetic monitoring, which is one component of anesthesia care. In certain rare or unusual circumstances, 1) some of these methods of monitoring may be clinically impractical, and 2) appropriate use of the described monitoring methods may fail to detect untoward clinical developments. Brief interruptions of continual† monitoring may be unavoidable. These standards are not intended for application to the care of the obstetrical patient in labor or in the conduct of pain management.
Standard II
Oxygenation
Objective
To ensure adequate oxygen concentration in the inspired gas and the blood during all anesthetics.
Methods
Ventilation
Methods
Circulation
Methods
† Note that “continual” is defined as “repeated regularly and frequently in steady rapid succession,” whereas “continuous” means “prolonged without any interruption at any time.”
* Under extenuating circumstances, the responsible anesthesiologist may waive the requirements marked with an asterisk (*); it is recommended that when this is done, it should be so stated (including the reasons) in a note in the patient’s medical record.
(Approved by the ASA House of Delegates on October 21, 1986, and Last Amended on October 25, 2005)
Indications for Careful Control of Fio2
Studies have implicated arterial oxygen tension as one of several variables linked to retinopathy of prematurity (ROP) in preterm infants (weighing less than 1300 g) whose retinas are immature (Flynn et al., 1992). The contribution of brief episodes of intraoperative hyperoxemia to the development of ROP remains unknown, and oxygen concentrations greater than necessary should be avoided in this vulnerable population. It is not known whether there is any real impact on outcome dependent on whether air or nitrous oxide is used as the balance gas in these patients. Given the potential for nitrous oxide to interfere with deoxyribonucleic acid (DNA) synthesis, it may be prudent to avoid this gas in the developing neonate, although there are no definitive data indicating that nitrous oxide is dangerous for these patients.
During airway laser surgery it is desirable to reduce the inspired oxygen concentration to less than 30% to minimize the risk of igniting the tracheal tube or any other combustible materials. Because nitrous oxide also supports combustion, it should not be used in these cases. Air can be used alone or with small amounts of additional oxygen. Helium is also a useful balance gas in these cases, because it does not support combustion and can improve the flow of inspired gases beyond any obstructions in the airway (Pashayan et al., 1988). Specially adapted anesthesia machines capable of delivering inspired carbon dioxide to achieve hypercarbia or increased inspired nitrogen to produce hypoxia may be indicated in the care of the neonate with specific types of congenital heart disease (Tabbutt et al., 2001). The important aspect of caring for these patients is to maintain the balance of pulmonary and systemic blood flow by controlling pulmonary vascular resistance. Both hypoxic oxygen concentrations and carbon dioxide can be used toward this end.
Anesthetic Vapor Delivery
All modern anesthesia machines deliver anesthetic vapor by vaporizing a controlled amount of liquid anesthetic and mixing it with the fresh gas entering the anesthesia circuit. The only exception is the Zeus anesthesia machine (Draeger Medical; Lubeck, Germany), which injects liquid anesthetic directly into the anesthesia circuit under closed-loop control. There are excellent sources of information on the details of vaporizer design; however, they are beyond the scope of this chapter (Dorsch and Dorsch, 2007).
Controlled Ventilation
The most important change to date in modern anesthesia machines is the ability to precisely deliver small tidal volumes accurately by compensating for breathing-circuit compliance and changes in fresh-gas flow. Newer anesthesia machines have ventilators that can precisely deliver small volumes at high rates (Stayer et al., 2000). Delivering small tidal volumes requires not only an accurate ventilator, but a means for compensating for the compliance of the breathing system and the interaction between fresh-gas flow and inspired tidal volume. Breathing-system compliance exists in any ventilator-circuit combination and results from the compression of gas within the breathing circuit as pressure builds in the circuit, as well as the elastic expansion of the breathing-circuit tubing. Breathing-system compliance is expressed as milliliters of volume that are taken up per centimeter of water pressure, and it is determined by the internal volume of the breathing system and circuit, as well as the elastic properties of the circuit. Longer breathing circuits have an increased compliance primarily because of the greater internal volume. To better understand the impact of breathing-circuit compliance on delivered tidal volume, consider a case where the compliance value is 1 mL/cm H2O and the inspiratory pressure is 20 cm H2O. In that case, 20 mL of tidal volume delivered by the ventilator will not reach the patient. If the desired tidal volume is small, for example 100 mL or less, the fraction that does not reach the patient because of circuit compliance becomes a significant fraction of the desired tidal volume.
To compensate for the compliance of the breathing system, the preuse checkout must be performed to allow the ventilator to measure the compliance of the breathing system and then compensate for that compliance factor during VCV. To use these ventilators effectively, it is essential that the preuse checkout procedures be followed with the breathing-circuit configuration that is to be used during the procedure (Fig. 10-5, A) (Bachiller et al., 2008). Different anesthesia-machine vendors have different approaches to eliminate the interaction between fresh-gas flow and tidal volume. Some anesthesia machines (e.g., Draeger Medical; Lubeck, Germany) use a decoupling valve between the ventilator and the fresh-gas inflow so that the valve closes during inspiration. Other anesthesia machine designs (e.g., General Electric; Madison, Wisconsin) compensate for the fresh-gas flow by using closed-loop feedback between the inspiratory flow sensor and the ventilator.
Assisted Spontaneous Ventilation
Another advantage of modern anesthesia ventilators for pediatric patients is the advent of pressure-supported modes of ventilation. These modes are specifically designed to allow for spontaneous ventilation when an endotracheal tube or supralaryngeal airway is in place and the associated work of breathing would be an impediment to effective spontaneous ventilation. In pediatric patients the work of breathing is a major problem, because small endotracheal tubes impose a significant resistance to inspiration, the ventilator circuits and valves add to the work of breathing, and the anesthetized patients have reduced inspiratory force. In general, long periods of unassisted spontaneous ventilation during anesthesia are undesirable and can result in progressive atelectasis. Supported ventilation is an excellent method for allowing a patient to breathe spontaneously, and it has been demonstrated to improve gas exchange during anesthesia (von Goedecke et al., 2005).
Three typical options for assisted spontaneous ventilation are listed as follows:
Warming Devices
Heat conservation is critical in the care of the newborn infant. A decrease of 2° C in the environmental temperature is sufficient to double the oxygen consumption of a full-term infant (Hill and Rahimtulla, 1965). To meet this increase in oxygen consumption, the neonate must double its minute ventilation. For a critically ill premature infant who is unable to mount this increase in ventilation, an oxygen debt and progressive lactic acidosis ensue (see Chapter 6, Thermoregulation: Physiology and Perioperative Disturbances). The rate of heat loss in newborn infants is four times that in adults because of a higher surface area-to-body weight ratio, increased curvature of body surfaces, and decreased insulation from skin and subcutaneous fat (Adamson and Towell, 1965). Special efforts must be made to maintain body temperature during anesthesia, especially in small infants undergoing operative procedures accompanied by large insensible heat losses.
Forced Warm-Air Device
The flow of warm air across a child’s skin produced by a forced-air warming blanket prevents heat loss to the environment and may even effectively warm patients via radiant shielding and convection. Of all devices, this is one of the most effective and should be used in all cases where hypothermia is a possibility (Sessler et al., 1991; Camus et al., 1993; Kurz et al., 1993). Forced-air systems inject warm air through a connecting hose into a quiltlike blanket that has small holes on one surface. Warm air escaping through these holes provides conductive and convective warming to the patient. Different sizes of blankets permit maximum coverage of specific body areas over a range of patient sizes. The most effective units direct flow toward areas with major blood vessels, like the chest, axillae, abdomen, and groin, where convective heating is most effective (Giesbrecht et al., 1994). Like overhead radiant warmers and circulating-water blankets, forced-air systems can produce burns and overheating when used inappropriately (Truell et al., 2000). Maximum air temperatures on these devices are designed to minimize the risk of burns. Ensuring that the tube carrying the warm air to the blanket does not touch the patient also decreases the likelihood of thermal injury. Furthermore, directing warm air from the hose without the blanket (“free-hosing”) has been associated with patient burns. Patients with compromised circulations are at a higher risk of burns even when the blanket is used correctly; the temperature should not be set to the highest setting in these patients, especially during prolonged surgery (Siddik-Sayyid et al., 2008). Blankets that are wet are ineffective at maintaining temperature and should be replaced (Lin et al., 2008).
Wrapping and Draping with Plastic Sheets
Because of their large surface area, infants have significant evaporative and radiant heat loss. Wrapping and draping the patient with special blankets is also effective in decreasing heat loss during prolonged surgery, especially when forced-air devices are impractical. Radiant heat loss can be decreased with the use of Webril cotton wrapping (Kendall Corporation; Boston, Massachusetts) covered with plastic bags over exposed extremities. For small infants with relatively large heads, cranial heat loss can be decreased up to 73% by wrapping the head in an insulated hat or plastic bag. This is significant for neonates, because their brains are responsible for 44% of their total heat production (Rowe et al., 1983). The use of reflective blankets, made from the material used in outdoor survival apparel (e.g., space blankets), is also effective in reducing heat loss (Bourke, et al., 1984). Cutaneous heat loss is proportional to the surface area of the patient, making the percentage of skin surface covered far more important than the region of the body covered or the type of material used for passive insulation (Sessler et al., 1991).
Humidification of Inspired Gases
Humidification of inspired gases, as noted previously, is an important technique for conserving heat in anesthetized patients. Because 12% to 14% of body heat is lost through the respiratory tract, the use of warm, humidified gases decreases the potential heat drain in addition to preventing damage to the ciliated cells of the tracheobronchial tree caused by dry gases (Clarke et al., 1954). The humidifier should be servocontrolled, with a temperature sensor close to the patient to automatically shut off the heater when the preset temperature equals the patient’s airway temperature, minimizing the risk of airway burns. Maximum temperature limits, causing automatic shutoff, should also be an integral aspect of the humidifier. Special anesthesia circuits with a heated wire in the expiratory limb minimizes condensation that can occur with humidifiers. HMEs (e.g., Humid-Vent) can provide 80% inspired humidity when 80 minutes of use is allowed to saturate the hygroscopic membrane (Bissonnette et al., 1989a, 1989b). Many HMEs are incorporated into breathing-system filters. These serve to minimize the passage of infectious materials to the breathing circuit and provide warmth and humidification of inhaled gases. The efficacy of various HME brands to perform these functions is variable. The internal volumes of these devices can range from 10 to 60 mL, and small volume filters are preferred in the neonatal population. HME filters may increase the work of breathing and dead space and should be accounted for when ventilating small children. Filtration efficacy is assessed by the penetrance of sodium chloride particles (0.1 to 0.3 mcm); tests in pediatric HME filters show that their filtration ability is less than in adult filters. Based on these findings the Centers for Disease Control (CDC) recommend changing the breathing circuit for each new case. Berry and Nolte (1991) examined the efficacy of the Pall HME filter in preventing bacterial contamination of the circle-breathing system in a laboratory model and found it to be an effective microbial barrier between the patient and the circle system. They concluded that when using the Pall HME filter the components beyond the filter may be reused. However the reuse of single-use equipment is not recommended and use is borne by the individual practitioner or institution. To date there has not been a reported case of infection transmission between patients related to anesthesia-breathing systems with filters in place.
Face Masks
The most common anesthesia face mask in use today is the plastic disposable type that contains an adjustable pneumatic cushion, which when inflated or deflated with air can be altered to conform to the shape of a child’s face. A variety of different manufacturers produce this type of face mask (Fig. 10-6). An alternative variety for use in pediatric patients is the Rendell-Baker-Soucek mask, which remains in use in many centers (Fig. 10-7
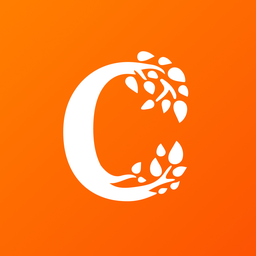
Full access? Get Clinical Tree
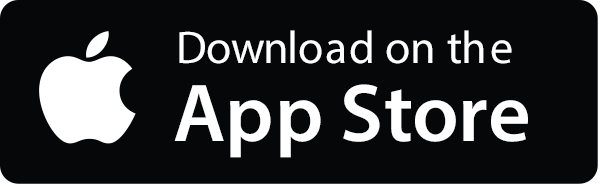
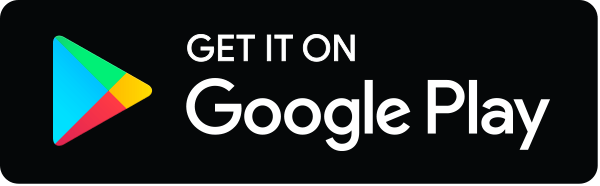