Epidural Neural Blockade
Bernadette T. Veering
Michael J. Cousins
Although techniques of epidural anesthesia do not offer the economy of drug dosage or degrees of blockade of spinal anesthesia, they are currently more versatile and better studied. No other neural blockade techniques are used as extensively in each of the fields of surgical anesthesia, obstetric anesthesia, and diagnosis and management of acute and chronic pain. Epidural blockade is also unique because of special features of the anatomic site of injection and the resultant diverse sites of action of the local anesthetic solution.
The most practical and widely used continuous method of neural blockade is spinal epidural blockade; pharmacokinetic data have helped to increase the efficacy and safety of epidural infusion techniques (see Chapter 40). Continuous caudal blockade has useful but limited applications, and continuous spinal anesthesia is beginning to be used more frequently. As indicated in Chapters 32 and 40, new developments in the understanding of pain conduction have extended the use of continuous epidural blockade to the administration of drugs that selectively block pain conduction, while leaving sensation, motor power, and sympathetic function essentially unchanged. The safety and reliability of spinal epidural catheter techniques, with the addition of bacterial filters, have permitted relief of acute pain (see Chapters 24, 40, and 43) and chronic pain (see Chapters 44 and 45) for many days, often with patients remaining ambulatory. This has heralded an even more vigorous and fruitful era of investigation and clinical application of epidural blockade than did the unprecedented development of the past 20 years.
Anatomy of Epidural Neural Blockade
Key anatomic features of the epidural space and of approaches to needle/catheter insertion are described in Chapter 9.
Epidural Pressures
The epidural space is identified traditionally by a sensation of negative pressure when entering the space. This negative pressure is believed to be created by tenting of the dura by the advancing needle, especially by blunt needles with side openings (1,2).
By using a closed measurement system that permitted continuous measurement of positive and negative pressure, lumbar epidural space pressures seem to be always positive with the needle stationary in the epidural space, except when the subarachnoid space was to be entered (Fig. 11-1) (3). At thoracic level, high negative epidural pressures up to -60 mm Hg were only observed at the moment of epidural puncture, equilibrating to a positive value within 90 seconds in both expiratory and inspiratory phases (4). This suggests that subsequent adaptation of the surrounding tissue results in restoration of the normal positive epidural pressure.
The lumbar epidural pressure increased with stimuli known to increase cerebrospinal fluid (CSF) pressure such as jugular venous compression, ventilation with carbon dioxide, and positive end expiratory pressure (5). This suggests that the lumbar epidural pressure is in equilibrium with the prevailing spinal CSF pressure (6).
Epidural injection of two different volumes of bupivacaine resulted in the same plateau pressure in both groups at the upper limit of normal for CSF pressure, suggesting a pressure-limiting feature in the epidural space (7).
Successful entry into the epidural space is sometimes followed by “drip back” when local anesthetic is subsequently injected, especially in elderly patients (8). This is probably due to a lower compliance of the epidural space, compared to that in younger patients.
In severe lung diseases such as emphysema, epidural negative pressure may be abolished, particularly if the patient is lying down (9). Any factor that increases abdominal pressure and/or occlusion of the inferior vena cava may distend the epidural veins and increase pressure in the lumbar epidural space. This results in only slight changes in the thoracic epidural space, particularly if the patient is sitting (6).
During labor, baseline lumbar epidural pressures are higher in women in the supine position compared with those in the lateral position. As labor progresses, baseline pressures increase with peaks of epidural pressure during each uterine contraction (10,11).
Coughing or a Valsalva maneuver increases both intrathoracic and intra-abdominal pressure, so that pressure in thoracic and lumbar epidural space increases (6), resulting in high positive pressures being recorded throughout the epidural space.
Comparison of patients having prior lumbar surgery to those who did not revealed a higher baseline lumbar epidural pressure in patients with previous lumbar surgery (12). However, additional pressure from epidural injections decays at a rate similar to that in patients who did not undergo operations. This suggests that the alteration induced by surgery is one of different initial condition, rather than a change in distensibility. On the other hand, the resistance to fluid injection in the epidural space was higher in patients with a diseased space, as the result of epidural arachnoiditis, compared with that of the normal space (13). Therefore, one should be careful with the
injection of fluid into parts of the epidural space that do not communicate freely with their surroundings.
injection of fluid into parts of the epidural space that do not communicate freely with their surroundings.
Physiologic Effects of Epidural Blockade
With currently available local anesthetic agents, spinal epidural neural blockade implies sympathetic blockade accompanied by somatic blockade, in the form of sensory and motor blockade alone or in combination. Although it is possible to avoid blockade of “peripheral” lumbar sympathetic fibers if only sacral segments are blocked by a caudal approach to the epidural space, spinal epidural blockade almost invariably results in some degree of sympathetic blockade. Some of the most important (but not all) of the physiologic effects of epidural blockade can be discussed in relation to either sympathetic blockade only of vasoconstrictor fibers (below T4) and/or of cardiac sympathetic fibers (T1–T4; Fig. 11-2). Many clinicians prefer to have a wide margin of safety if they are intent on avoiding major sympathetic blockade. Thus, they aim to restrict the level of analgesia to T10. Studies discussed in Chapter 38, however, indicate that the level of sympathetic block with epidural anesthesia may be lower than the level of sensory block and more incomplete in terms of the quality of block (14). This concept of sympathetic blockade is still a practical approach in considering the physiologic effects of epidural blockade because inguinal, perineal, urologic, and lower limb surgical procedures can be carried out with blockade to T10 or lower, which at most will produce only a “peripheral” sympathetic blockade. However, lower abdominal surgery (such as appendectomy, gynecologic surgery, and cesarean section) necessitates blockade to T4. Thus, the most frequent use of epidural block will be to either the T4 or T10 level. Occasionally, a T1 level is needed for chest injury or thoracotomy. We will consider the cardiovascular
effects of epidural block with respect to the degree of sympathetic block (and its effects) with sensory blockade to T1, T4, or T10.
effects of epidural block with respect to the degree of sympathetic block (and its effects) with sensory blockade to T1, T4, or T10.
Subtler and somewhat more indirect is the reduced input to the central nervous system (CNS), which accompanies various levels of sensory blockade. This “deafferentation” has long been thought capable of exerting a “protective” effect that reduces the efferent neurohumoral response to surgical stimulation or trauma. Objective data have now become available to support this hypothesis (see Chapters 6 and 7).
Finally, it is important to remember that extensive epidural blockade often requires large doses of local anesthetic (with or without epinephrine) (Fig. 11-3). Large doses themselves may cause physiologic changes as a result of the direct pharmacologic effects of circulating blood concentrations. These are an inevitable outcome of vascular absorption from the epidural space. Because of the unique epidural venous system (see Chapter 9), direct intravascular injection may result in the rapid attainment of high concentrations of local anesthetic in the brain and/or heart, with the potential for convulsions and/or sudden depression of cardiac output (see Chapters 3,4,5). Also, important changes in vagal tone accompany sympathetic block (Fig. 11-4). The various mechanisms for physiologic effects of epidural block are summarized in Table 11-1.
Cardiovascular Effects of Epidural Blockade
Block of sympathetic innervation accounts for the cardiovascular responses to epidural anesthesia. The preganglionic sympathetic innervation plays an important role in regulating regional blood flow. Blockade of sympathetic nerve fibers and resulting loss of vasomotor tone will therefore cause considerable changes in blood flow to various organs, depending on the level achieved (Fig. 11-2) (15). Postganglionic sympathetic nerves are important in controlling cardiac function and vascular tone. High thoracic epidural anesthesia (TEA), from the first to fifth thoracic segments, blocks the cardiac afferent and efferent sympathetic fibers, with loss of chronotropic and inotropic drive to the myocardium (16). Epidural anesthesia that is restricted to the level of the low thoracic and lumbar region (T5–L4) results in a peripheral sympathetic blockade with vascular dilatation in the pelvis and lower limbs.
Cardiovascular depression may occur with both epidural and subarachnoid blockade (Fig. 11-3) and is at least partly related to the level of sympathetic blockade (Fig. 11-2, see also Chapter 10) (17,18).
Also, vascular absorption of local anesthetic and vasoconstrictor may result in significant hemodynamic changes after epidural but not after subarachnoid blockade; the reason for this lies predominantly in the much larger doses of drugs used in epidural blockade and in the proximity of the large epidural veins, which, owing to their anatomy, have considerable potential for rapid transport of drug to the heart (Table 11-2) and CNS.
When comparing the physiologic effects of spinal and epidural anesthesia, it is relevant to consider if spinal anesthesia does result in a more complete sympathetic blockade than does epidural anesthesia. A possible approach to investigate the intensity of sympathetic block is to measure catecholamine response to immersion of a limb in cold water (cold pressor test). Plasma catecholamines and hemodynamic responses were similar following both techniques, indicating that spinal anesthesia did not result in a more profound attenuation of the sympathetic response than did epidural anesthesia (19).a
High Thoracic Segmental Epidural Anesthesia (T1–T5)
Epidural blockade of T1–T5 segments has the following effects on cardiac sympathetic activity: (a) blockade of segmental cardiac reflexes in segments T1–T4, (b) blockade of outflow from vasomotor center to cardiac sympathetic fibers (T1–T4), and (c) vasoconstrictor nerve blockade in head, neck, and upper limbs. Denervation of preganglionic cardiac accelerator fibers leaving the cord at T1–T5 results in minimal vasodilatory consequences.
However, changes in heart rate, LV function, and myocardial oxygen demand may occur because of high thoracic epidural blockade and are discussed next.
Heart Rate
During cardiac sympathetic denervation, parasympathetic cardiovascular responses, including those involved in baroreflexes may dominate.
A high TEA covering the cardiac segments (T1–T4) produces small but significant reductions in heart rate (20,21,22). Because changes in cardiac rate are known to be controlled chiefly by the balance of sympathetic and parasympathetic tone at any moment, it must be assumed that parasympathetic tone is reduced to almost the same degree as sympathetic tone to maintain heart rate at a normal or near normal level (21,22) (Table 11-3). Whether the sympathetic nervous system functions as a direct cardioaccelerator or indirectly by modifying the parasympathetic tone needs to be clarified.
Left Ventricular Function and Myocardial Oxygen Demand
Blockade from T1 to T5 influences the contractility of the LV. However, discrepancies exist among studies that have evaluated the influence of high TEA on the LV contractility. Some studies demonstrated that blockade from T1 to T5 is associated with a negative inotropic effect on the LV (23,24). The LV contractility decreased with an approximate 15% reduction of stroke volume in patients with TEA from C5 to T5. On the other hand, high segmental TEA did not produce a reduction in LV function, as measured by the suprasternal Doppler method (25) or by echocardiography (26) in patients scheduled for elective noncardiac surgery. Both studies documented a small but statistically significant decrease in heart rate, a minor decrease in mean arterial pressure, and essentially no change in cardiac index. A study in healthy volunteers receiving thoracic epidural blockade from C7 to T6 produced only a minor decrease in systolic blood pressure and no significant change in cardiac output (27).
In contrast in patients with coronary artery disease (CAD) subjected to physical stress, high TEA improves global and regional LV function while diminishing associated changes in ST segments (28). The most likely explanation for the improvements in LV global and regional function during TEA and exercise is that TEA beneficially affects the oxygen supply-to-demand ratio in the ischemic area mainly by reducing myocardial oxygen demand. The LV diastolic function appears to improve in resting patients with CAD after high TEA (29).
Effects of Thoracic Epidural Blockade on the Ischemic Hearta
With a high TEA, the cardiac sympathetic nerves are blocked and this will have an impact on the myocardium (30).
Following high TEA in an animal model the size of experimentally induced myocardial infarcts was reduced after experimental coronary occlusion (31). In another animal study, it was demonstrated that the endocardial-to-epicardial blood flow ratio increased, and this may have decreased ischemic injury in association with TEA (32). In addition, the incidence of ischemia-induced malignant arrhythmias in anesthetized rats was reduced by high TEA (33). Thus, high TEA exerts a cardioprotective effect during experimental myocardial ischemia by improving the myocardial oxygen supply-to-demand ratio.
Table 11-1 Mechanisms for physiologic effects of epidural analgesia | ||||||||||||||||||||||||||||||||
---|---|---|---|---|---|---|---|---|---|---|---|---|---|---|---|---|---|---|---|---|---|---|---|---|---|---|---|---|---|---|---|---|
|
High TEA in humans improves an ischemia-induced LV dysfunction; reduces electrocardiographic, echocardiographic, and angiographic signs of coronary insufficiency; decreases the incidence of arrhythmias; and provides relief of ischemic chest pain (34,35,36). Furthermore, it has been reported that high TEA improves ischemia-induced LV global and regional wall motion abnormalities while diminishing associated changes in ST segments in patients with CAD under physical stress (28). This is probably related to the decrease in LV afterload per se improving regional wall motion (see also Chapter 7).
High TEA has probably no influence upon the autoregulation of coronary resistance vessels; it should therefore not adversely affect regional blood flow distribution in patients with CAD with a pattern that provides a basis for coronary steal (i.e., maldistribution of coronary blood flow) (37,38). In addition, in patients with multivessel ischemic heart disease, TEA partly normalizes the myocardial blood flow response to sympathetic stimulation (39).
Thoracic Epidural Anesthesia (T1–T12)
An epidural block from T1 to T12 (using a mid to high thoracic catheter) or even involving the upper lumbar segments is associated with extensive sympathetic block. Consequently, hypotension will occur, partly by the block’s cardio depressant action and consequent reduction in systemic vascular resistance. Such block decreases preload and afterload as a result of increased venous capacitance and redistribution of blood volume in the affected dilated splanchnic vascular beds (T6–L1). Denervation of the splanchnic fibers leads to vasodilatation of the visceral (splanchnic) venous bed, resulting in pooling of blood in the gut and abdominal viscera. The splanchnic veins produce the majority of the total systemic reflex capacitance changes and account for almost all of the reflex venoconstriction that buffers the systemic circulatory volume changes (40).
The depressant effects are partly counteracted by vasoconstriction in the diminished remaining innervated body regions (41). This increased efferent sympathetic activity is mediated predominantly (by means of the baroreceptors) by those sympathetic vasoconstrictor nerves (T1–T5) that remain unblocked and by circulating catecholamines released from the adrenal medulla, owing to increased activity in any unblocked fibers in the splanchnic nerves (T6–L1). Although blood vessels in some viscera, such as the kidney, appear to be more responsive to direct neural stimuli in other vascular beds, both neural and hormonal influences have major effects, although at different levels of the vasculature. Major arterioles respond mostly to neural stimuli, whereas small arterioles and venules near the capillary bed respond predominantly to circulating catecholamines. Thus, while any splanchnic fibers remain unblocked, there is a potential for vasoconstrictor activity below (as well as above)
the level of blockade, through release of catecholamines from the adrenal medulla. Finally, the ability of precapillary sphincters to achieve autoregulation within a short time of cessation of neural activity (42) provides a further mechanism for regaining vascular tone and minimizing vascular pooling below the level of blockade.
the level of blockade, through release of catecholamines from the adrenal medulla. Finally, the ability of precapillary sphincters to achieve autoregulation within a short time of cessation of neural activity (42) provides a further mechanism for regaining vascular tone and minimizing vascular pooling below the level of blockade.
Catecholamine response to immersion of a limb in cold water (cold pressor test) revealed that epidural anesthesia with a sensory level to T1 did not produce complete sympathectomy regardless of the local anesthetic used, since plasma concentrations of catecholamines were nearly unchanged (43). Blockade of preganglionic sympathetic fibers innervating the adrenal medulla and innervating peripheral sympathetic fibers appeared to be incomplete, even during quite extensive epidural anesthesia to C8 (44).
Epidural anesthesia is probably associated with a reduction in sympathetic neural transmission rather than a complete blockade of sympathetic fibers.
The potential magnitude of cardiovascular changes has been documented in unmedicated volunteers with a blockade of C5–S5; that is, complete blockade of cardio-accelerator fibers and adrenal medullary catecholamine secretion (17,45). The mean arterial blood pressure was reduced approximately 20%, with a similar reduction in total peripheral resistance, minimal changes in heart rate, and a rise central venous pressure (26%). This small change in heart rate gives a deceptive picture of cardiac sympathetic activity, since Otton and Wilson have shown that blockade of T1–T4 alone produced an increase in central venous pressure (CVP) without an increase in stroke volume output of the heart (27).
Table 11-2 Cardiovascular effects of epidural blockade | ||||||||||||||||||||||||||||||||||||||||||||||||||||||||
---|---|---|---|---|---|---|---|---|---|---|---|---|---|---|---|---|---|---|---|---|---|---|---|---|---|---|---|---|---|---|---|---|---|---|---|---|---|---|---|---|---|---|---|---|---|---|---|---|---|---|---|---|---|---|---|---|
|
Mid Thoracic to Lumbar Epidural Anesthesia (T5–S5)
Epidural blockade that is restricted to the level of the low thoracic and lumbar region (T5–L4) results in a “peripheral” sympathetic blockade with vascular dilatation in the pelvis and lower limbs; if all splanchnic fibers are blocked (T6–L1), then pooling of blood in the gut and abdominal viscera also may occur (Table 11-2). This peripheral blockade has been demonstrated by measurements of large increases in lower limb blood flow owing to arteriolar vasodilation (46) and pooling of blood in the venous capacitance vessels (47). Because the latter contain 80% of blood volume, venodilatation has a potential for dramatic changes in venous return, reduction in right atrial pressure, and reduced cardiac output. The decrease in venous return has been shown to result in increased cardiac vagal tone. This explains why heart rate remains unchanged or decreased despite hypotension and activation of cardiac sympathetic accelerator fibers (48).
While any splanchnic fibers remain unblocked, there is a potential for vasoconstrictor activity below (as well as above) the level of blockade, through release of catecholamines from the adrenal medulla. Increased activity in cardiac sympathetic fibers (T1–T4) may result in increased cardiac contractility and increased heart rate; similar effects are produced by increased levels of circulating catecholamines. Evidence that the latter
are important in maintaining homeostasis in some clinical situations is provided by the surprisingly small changes in heart rate and cardiac output (-16%) with blockade of C5–T4, but with splanchnic fibers to the adrenal medulla (T6–L1) intact (27).
are important in maintaining homeostasis in some clinical situations is provided by the surprisingly small changes in heart rate and cardiac output (-16%) with blockade of C5–T4, but with splanchnic fibers to the adrenal medulla (T6–L1) intact (27).
Table 11-3 Danger Signals: Cardiovascular Effects of Epidural Block | |
---|---|
Distribution of blood to the splanchnic area was studied by Arndt et al. in healthy young volunteers after injection of 20 mL of plain 2% lidocaine into the lumbar region. Splanchnic blood volume decreased as assessed by distribution of radiolabeled red cells. Radioactivity also decreased in thorax and upper limbs, indicating compensatory vasoconstriction (49).
Low Thoracic to Lumbar Epidural Anesthesia (T10–S5)
Lumbar epidural anesthesia (LEA) with a sympathetic blockade below T10 results in minimal vasodilatory consequences because fewer vasoconstrictor fibers are included, and neither the
splanchnic nerves nor the nerve supply to the adrenal medulla are affected. Since muscle veins lack sympathetic innervation, venodilatation of the extremities is limited to the skin, and so minimal capacitance increase results from blocks of the lower extremities (50). A block below L3 does not affect the sympathetic system, and the hemodynamic upset is minimal.
splanchnic nerves nor the nerve supply to the adrenal medulla are affected. Since muscle veins lack sympathetic innervation, venodilatation of the extremities is limited to the skin, and so minimal capacitance increase results from blocks of the lower extremities (50). A block below L3 does not affect the sympathetic system, and the hemodynamic upset is minimal.
Lumbar epidural anesthesia with a sympathetic blockade extending to the lower segments may occasionally be associated with profound bradycardia and circulatory collapse without any obvious precipitating event. This issue is discussed in the section on central volume depletion.
Absorbed Local Anesthetics
Relatively large amounts of local anesthetic solutions are required to achieve satisfactory epidural anesthesia. Local anesthetics exhibit a direct biphasic action on vascular smooth muscle, depending upon the local anesthetic agent, its concentration and stereochemical configuration, the type of blood vessel (capacitance or resistance), and preexisting vascular tone (see Chapter 3). At low concentrations, local anesthetics cause vasoconstriction. At concentrations used for epidural anesthesia, vessels tend to vasodilatate (51). At high concentrations of lidocaine, Bonica observed stimulatory effects on the circulation (17). Such stimulation was thought to be caused by a central effect of lidocaine enhancing sympathetic activity by means of remaining cardiac sympathetic fibers. Generally, the systemic effects produced by concentrations of local anesthetics in blood that are associated with correctly performed epidural block have little, if any, clinical significance (Table 11-2).
Epinephrine-Containing Solutions
Epinephrine injected alone into the epidural space (i.e., 100 μg; 20 mL of 5 μg/mL) will be absorbed slowly because of its local vasoconstrictor effect. The systemic effects of achieved epinephrine blood levels are β-adrenergic; that is, a moderate increase in heart rate, increased cardiac output, decreased peripheral resistance, and unchanged mean arterial pressure. The vasodilatation results in augmentation of the vasodilatation induced by the sympathetic denervation associated with epidural anesthesia, so that the total effect in epidural block is likely to be a larger decrease in mean arterial pressure than if solutions of plain local anesthetic are used. The early cardiovascular changes observed with absorbed epinephrine are, however, transient. Therefore, the prolonged cardiovascular changes seen with local anesthetics containing epinephrine probably relate to sympathetic blockade. The most likely explanations for the more pronounced cardiovascular effects of epinephrine-containing local anesthetic solutions appear to be as follows:
Systemic absorption of epinephrine. β-Adrenergic effects on the heart, resulting in increased heart rate and cardiac output; peripheral vascular β-adrenergic effects, resulting in further vasodilatation within the area of sympathetic block; and antagonism of compensatory vasoconstrictor responses outside the area of blockade. Thus, total peripheral resistance falls, and mean arterial blood pressure is reduced to a degree comparable to that seen with equivalent levels of subarachnoid blockade (Fig. 11-3).
More intense neural penetration or more extensive spread of neural blockade, resulting in more reliable sympathetic block.
Hypovolemia and Epidural Block
Bonica demonstrated in healthy volunteers that epidural block to T5 was associated with major reductions in heart rate, cardiac output, and mean arterial pressure in the presence of hypovolemia in comparison to mild cardiovascular changes at normovolemia (17,45,52). Cardiovascular homeostasis was better maintained with epinephrine–lidocaine blockade. However, marked reductions in mean arterial blood pressure still occurred (Fig. 11-5). Because cardiac sympathetic fibers were thought not to be blocked in these patients, an explanation was sought for the large reductions in heart rate and cardiac output. The abrupt bradycardia resulting in cardiovascular collapse in human subjects is probably a sudden vagal response to marked reductions in venous return (53,54,55,56) (Tables 11-3 and 11-4). The mechanism is discussed in detail in the next section, on central volume depletion.
The less pronounced cardiovascular depression in hypovolemic subjects receiving epidural block with lidocaine–epinephrine is probably due to an increase in heart rate due to absorption of epinephrine. This may have protected the heart from increases in vagal activity although it has been noted that a high level of sympathetic activity may accentuate cholinergic effects in patients with poor venous return (56). It is also possible that peak arterial blood concentrations of lidocaine were higher after plain lidocaine in patients with hypovolemia, owing to decreased cardiac output and thus a smaller volume of distribution (see Chapter 3). In this situation, the myocardium receives a larger percentage of cardiac output and thus is potentially exposed to higher concentrations of local anesthetics (see Chapter 5). Any coexistent hypercapnia or acidosis would tend to accentuate the depressant effects of local anesthetic on the myocardium (see Chapter 3). The moral of Bonica’s study is clear (18): Epidural block should be avoided or used with great care in patients with uncorrected hypovolemia or in any other patient in whom venous return is markedly impaired (e.g., patients with large intra-abdominal masses in whom the pressure of the mass on the vena cava cannot be relieved before blockade).
Central Volume Depletion
Epidural anesthesia with and without involvement of cardiac segments may be associated with profound bradycardia and, in some patients, with transient cardiac arrest without any obvious precipitating event (57) (Tables 11-3 and 11-4). Apparently, responses by vagal reflex predominate, associated with echocardiographic evidence of smaller LV chamber size (58). This bradycardia seems to be mediated via the LV mechanoreceptors, activated by a reduction in end-systolic volume, occurring as a result of decrease in venous return. A vago-vagal reflex (the Bezold-Jarisch reflex) elicited from and returning to the heart is thereby activated and is likely a protective reflex (59,60). This reflex slowing should allow time for more complete filling of the heart. So, hypovolemia can precipitate not only classic vagal symptoms, but also full cardiac arrest in healthy patients. Vagal activation with bradycardia is thus a protective reflex that prevents the heart from contracting when relatively empty. Possible CNS hypoperfusion and an obtunded response to hypoxia from epidural block may intensify the bradycardic response to volume depletion (61).
Recent animal research demonstrated the cardiodepressor response to be mediated from regions in the brainstem triggering the sympathoinhibitory reflex to central hypovolemia (62).
Epidural Anesthesia and General Anesthesia
Thoracic epidural anesthesia associated with light general anesthesia is increasingly used for upper abdominal surgery and for
major vascular surgery. Lumbar epidural anesthesia combined with general anesthesia is commonly used for prolonged major lower abdominal and pelvic surgery. Combined epidural and general anesthesia offers the advantage of a rapid and less painful recovery. This combination technique may result in a greater degree of hypotension than with each technique alone (63,64).
major vascular surgery. Lumbar epidural anesthesia combined with general anesthesia is commonly used for prolonged major lower abdominal and pelvic surgery. Combined epidural and general anesthesia offers the advantage of a rapid and less painful recovery. This combination technique may result in a greater degree of hypotension than with each technique alone (63,64).
Table 11-4 Vagal and sympathetic activity: effects on heart rate | |
---|---|
Such hypotension arises mainly from a decrease in venous return and is also due to attenuation of the compensatory vasoconstriction of nonanesthetized sympathetic tone via central depressant effects on vasomotor center (65,66). Obviously, the greatest concern for the consequences of hypotension is in patients with CAD. Thoracic epidural anesthesia plus light general anesthesia did not worsen (or improve) ventricular wall motion or induce myocardial ischemia, suggesting that myocardial oxygen balance was maintained (67). On the other hand, LEA with general anesthesia may cause impairment of segmental ventricular wall motion (SWM), indicating myocardial ischemia (68). In comparing these two studies, it would seem that the block of efferent sympathetic innervation to the heart that occurs with TEA but not with lumbar epidural blockade has beneficial effects.
In clinical practice, ephedrine and phenylephrine are the two agents that are commonly recommended to treat hypotension during epidural anesthesia associated with general anesthesia (69). Ephedrine appears to be the drug of choice to restore blood pressure under TEA associated with general anesthesia (70,71), since LV function was not compromised.
Phenylephrine seems to be the drug of choice to restore mean arterial pressure in patients who were moderately hypotensive during LEA combined with general anesthesia (71).
In summary, it appears that light general anesthesia can be safely combined with epidural block to the level of T5 in healthy patients. Use of a slight head-down tilt to maintain venous return and small incremental doses of atropine to maintain heart rate at approximately 90 beats per minute are recommended to treat moderate hypotension during combined lumbar epidural and general anesthesia. If additional cardiovascular support is required, ephedrine should be used depending on each patient’s cardiovascular status.
Important Aspects of Venous Return and Epidural Blockade
As indicated in Tables 11-3 and 11-4 and in Figures 11-4 and 11-5, reduced venous return may play a dominant role in initiating sudden reductions in cardiac rate, which should be viewed as a danger signal that venous return is markedly reduced and oxygenation of the myocardium is at risk. Obstruction to venous return, by whatever means, must be avoided in patients being given epidural block. If postural changes are added to obstruction in the presence of the increased venous capacitance of epidural block, serious impairment of venous return will follow. In addition, pressure in epidural veins will rise owing to channeling of blood from the pelvis by way of the alternative route of the vertebral venous plexus and azygos vein to the right atrium; this has important consequences for increased spread of segmental analgesia and may impair arterial blood flow to the spinal cord (72).
Situations in which venous return may be compromised may be summarized as follows:
Supine hypotensive syndrome in pregnancy resulting from uterine compression of the vena cava is accentuated by increased venous capacitance owing to sympathetic block of epidural analgesia and postural changes favoring pooling of blood in the lower limbs (73,74).
Uterine contraction during labor in supine position may cause compression. Mean brachial arterial pressure may be maintained at deceptively normal levels because of simultaneous compression of vena cava and aorta (Poseiro effect). However, mean femoral arterial pressure drops precipitously, as does uterine blood flow (75). These effects are accentuated by epidural block if the patient is allowed to remain supine.
Intestinal obstruction, ascites, and large intra-abdominal tumors may compress the vena cava at three main sites: (a) below the liver, owing to abdominal distention, by intestinal obstruction (76), or by ascites (77) (this site is also commonly occluded by overenthusiastic retraction or by abdominal packs during upper abdominal surgery); (b) in the upper lumbar region, by large intra-abdominal tumors (including within the uterus); and (c) at the pelvic brim, by stretching of the iliac vessels owing to extreme backward tilting of the pelvis. This is sometimes an accompaniment of later pregnancy and may also result from extreme lordotic posturing on the operating table. The “extended lordotic posture” may occlude the vena cava below the liver as well—with potential for venous congestion in the kidney and resultant proteinuria (78).
The most common causes of vena caval obstruction in surgical applications of epidural block are poor positioning, heavy-handed retraction, and incorrect use of abdominal packs. Extreme positions, such as the jackknife prone, lateral “kidney,” and hyperflexed lithotomy, should be avoided in association with any anesthetic technique and with epidural block in particular (73,79). Whenever possible, caval obstruction should be relieved before epidural block or carefully avoided after epidural block. If it occurs and cannot be corrected for a period of time, venous return may be assisted by restoring venous capacitance to normal levels by using carefully titrated doses of ephedrine (5–10 mg) intravenously. In some patients with large abdominal tumors, both the aorta and vena cava may be partially obstructed, but with maintenance of sufficient venous return to keep mean arterial pressure normal, with a partly occluded aorta. Sudden relief of the aortic obstruction as the tumor is removed may cause a precipitous fall in blood pressure owing to the reactive hyperemia below the level of obstruction. This situation may be avoided by ensuring adequate hydration and perhaps by using appropriate amounts of colloid before tumor removal. Also, one should be prepared to use small doses of ephedrine until reactive hyperemia subsides.
Epidural Blockade and Reduction of Blood Loss
Although initial emphasis on methods to reduce operative blood loss focused on reduction of arterial blood pressure, it was also well known that position played an important part (80). There has been a gradual recognition of the importance of avoidance of venous obstruction and the use of position in combination with sympathetic blockade to aid venous pooling away from the operative site. Thus, although epidural blockade has been used to produce hypotension and, in turn, control operative blood loss (81,82), others have found that blood loss can be reduced without the levels of hypotension commonly required if general anesthesia and hypotensive drugs are used (83,84). Keith deliberately avoided arterial hypotension in a randomized prospective study of blood loss using epidural or general anesthesia for surgery for total hip replacement (83). Blood loss was determined intraoperatively by a colorimetric technique and postoperatively by closed suction drains. Patients receiving epidural block had operative blood losses that were half those associated with general anesthesia. In contrast, there was no difference in postoperative blood losses between the two groups. Other studies reported a reduction in blood loss by 30% to 40% if epidural block is used for hip surgery (85,86). Thus, it appears that epidural block may reduce operative blood loss by factors other than a mild reduction in arterial blood pressure, increased venous capacitance (47), and the use of appropriate position. Additional factors may include the prevention of high venous pressure in response to sympathetic activity resulting from pain (87), avoidance of “reactive arterial hypertension,” and avoidance of increased airway pressure with resultant effects on venous pressure.
Function of Hollow Viscera After Epidural Blockade
The Bladder
One of the most commonly observed sequels of lumbar epidural block is temporary atonia of the bladder owing to blockade of sacral segments S2–S4. This is similar to lower motor neuron lesions in which bladder sensation is lost. Fortunately, this type of effect after epidural blockade is usually short-lived and causes no or minimal increases in postblock bladder dysfunction (88). However, careful postepidural monitoring of bladder function is advised (e.g., by postvoid bladder scans).
When continuous epidural techniques are used, however, catheterization of the bladder may be necessary (89). On the other hand, segmental thoracic epidural block (e.g., T5–L1) may spare the sacral segments and thus leave bladder sensation intact. In addition, relief of severe abdominal pain by epidural block from T5 to L1 may prevent reflex sympathetic activity (via T12–L1 spinal segments), which increases bladder sphincter tone and may predispose to acute retention.
The Gut
Epidural block extending from T6 to L1 effectively denervates the splanchnic sympathetic supply to the abdominal viscera (see Chapter 39, Fig. 39-1). The sympathetic blockade results in a small contracted gut owing to parasympathetic dominance.
Colorectal surgery is associated with postoperative ileus, which contributes to delayed discharges. Blockade of nociceptive afferent and sympathetic efferent nerves are believed to be key initiators of ileus. Thoracic epidural anesthesia with postoperative thoracic epidural analgesia has been shown to shorten the time to first bowel movement (90). The duration of postoperative colonic ileus is shortened with TEA using local anesthetics (91,92), compared with parental and epidural opioids (93). The main mechanism appears to be a block of the nociceptive afferent fibres and the thoracolumbar nociceptive efferent fibres with unopposed parasympathetic efferent fibers (90).
Lumbar epidural blockade appears to be not effective in inhibiting bowel motility (94). Perioperative intestinal hypoperfusion is a major contributing factor leading to organ dysfunction. Thoracic epidural anesthesia during and after major surgery has been shown to protect the gut from decreased microvascular perfusion and to improve the mucosal blood flow, even under conditions of decreased perfusion pressure (95,96).
Blood flow in the intestine, as assessed by laser Doppler flowmetry, has been shown to increase in patients undergoing colon surgery (97) and during experimental gastric tube formation (98) under epidural anesthesia. This increase may be beneficial and may contribute to the healing of the gut anastomoses if epidural local anesthetic agents are used for postoperative analgesia (see also Chapters 6 and 7).
Thus, epidural anesthesia with local anesthetics seems to be the best method for relieving pain after gastrointestinal surgery because of its minimal effects on gastric emptying, stimulating effects on bowel motility, and possibly beneficial effects on the integrity of bowel anastomoses.
Thermoregulation and Shivering
Hypothermia (a decrease in core temperature) is common in patients undergoing surgery with epidural anesthesia and is thought to result from heat loss to the cold environment due to sympathectomy-induced vasodilatation. The normal process by which thermoregulation usually minimizes intraoperative core temperature is prevented, since epidural anesthesia directly inhibits vasoconstriction in the analgesic dermatomes.
Hypothermia following epidural injection of local anesthetic solution may result in part from redistribution of heat from central to peripheral regions (99,100,101). Warming the body via the skin for 2 hours before inducing epidural anesthesia raised the skin temperature (but not the core temperature) and helped prevent hypothermia during the induction of epidural anesthesia (99).
With epidural anesthesia, shivering-like tremors occur in approximately 30% of patients (102). The decrease in core temperature triggers thermoregulatory vasoconstriction and shivering above the level of epidural anesthesia (100,101).
Cold solutions injected into the epidural space may directly affect thermosensitive structures within the spinal cord to cause shivering during epidural anesthesia in pregnant subjects (103,104) but not in nonpregnant subjects (101). This suggests that pregnancy may enhance the contribution of spinal thermoregulatory input.
Injection of either epidural meperidine (25 mg) or epidural fentanyl (50 μg) has been reported to abolish shivering during epidural local analgesia in a high percentage of patients in labor (105,106) and during cesarean section (107).
In summary, tremor during epidural anesthesia is due to normal thermoregulatory shivering, which results largely from central hypothermia and is preceded by peripheral vasoconstriction above the level of sympathetic blockade.
Neuroendocrine Effects of Epidural Blockade
Surgical stress is associated with a variety of changes in endocrine and metabolic function, including protein metabolism, leading to a state of negative nitrogen balance in the postoperative period. Most of the surgically induced endocrine and metabolic changes are abolished by an appropriate level of sensory blockade produced by regional anesthesia. If the level of anesthesia extends from T4 to S5, and if the epidural blockade is initiated before the onset of surgery, epidural anesthesia for lower abdominal procedures and operations on the lower extremities may completely abolish the hormonal and metabolic response. However, epidural anesthesia is less efficient in decreasing the surgical stress response to major upper abdominal and thoracic procedures (108). This is probably due to the inability of the epidural anesthetic to completely block all nociceptive afferent pathways (109). Also, evidence suggests that the afferent neural impulses as well as humoral factors, such as cytokines, which are released from the site of injury, may initiate the stress response in the hypothalamus during upper abdominal surgery (110). Combinations of local anesthetics and opioids administered epidurally in the postoperative period provide more complete blunting of the neuroendocrine response than do opioids alone (111).
The metabolic alterations observed during surgery and the modifying effect of intra- and postoperative epidural anesthesia–analgesia on the metabolic stress response to surgery are discussed in detail in Chapter 6.
Vasoactive Hormones
The arginine-vasopressin (AVP) system and the renin-angiotensin system (RAS) play an important role in maintaining arterial blood pressure under conditions in which the sympathetic system is impaired (112,113). In an animal model, vasopressin concentrations increased during high TEA, most likely to compensate for decreased cardiac filling and/or arterial blood pressure when sympathoadrenal responses are impaired (114).
However, in humans, both renin and vasopressin support blood pressure and prevent severe hypotension following epidural anesthesia (115).
In nonpremedicated patients, active renin and vasopressin concentrations were measured in response to sodium nitroprusside (SNP)-induced arterial hypotension both before and during sympathetic blockade by epidural anesthesia (116). Sodium nitroprusside-induced hypotension was associated with increased plasma renin concentrations with the sympathetic system intact, but not during sympathetic blockade by TEA. Vasopressin plasma concentrations increased during SNP-induced hypotension in the presence of widespread epidural sympathetic blockade by segmental thoracic epidural blockade from T1 to T11, but not with the sympathetic system intact (Fig. 11-6B). During sympathetic blockade by epidural anesthesia, the responses to the second hypotensive challenge were significantly different from the first: Heart rate increased by less than half of the increase following the first challenge (Fig. 11-6A).
In summary, sympathetic blockade by TEA abolished the increase in renin activity in response to arterial hypotension. This indicates that, in humans, the renal sympathetic system probably plays a key role in mediating renin release in response to hypotension. In addition, TEA activates the vasopressin system in response to hypotension.
Understanding the role of these endogenous vasopressor systems in maintaining blood pressure after epidural blockade in healthy subjects may help to explain why certain high-risk patients become hypotensive during high TEA.
Effects of Epidural Blockade on Respiration
Two important questions concerning respiration and epidural blockade require an answer: Does epidural block interfere with respiration? Is the ability to cough impaired?
The following aspects of epidural blockade may influence respiration:
Sensory (“afferent”) neural blockade reduces nociceptive afferent drive to respiratory center
Motor (“efferent”) neural blockade of intercostal muscles, abdominal muscles, and diaphragm (rarely)
Sympathetic neural blockade with resultant changes in cardiac output and pulmonary blood flow
Vagal dominance in the presence of complete sympathetic blockade
Systematically absorbed epinephrine and local anesthetic have effects upon:
Respiratory control center in midbrain and chemoreceptors in medulla and carotid bodies
Myoneural junction
Metabolism of succinylcholine in serum
The potential for phrenic (C3–C5) palsy is extremely low with epidural block, since even blockade to T1 produces motor blockade to only the T4–T5 level. The only exception may be intentional epidural block at the cervical level or inadvertent epidural block during interscalene brachial plexus block (see Chapter 13).
Respiratory arrest during high epidural blockade is not usually the result of the effects of sensory or motor blockade, nor is it due to depressant effects of local anesthetic in the CSF; the concentrations attained in the brain by means of this route are insufficient to depress neuronal activity unless gross overdosage is administered (117). The most common causes of the rare instances of respiratory arrest associated with epidural block are extensive sympathetic blockade, reduced cardiac output, and reduced oxygen delivery to the CNS. It cannot be overemphasized that meticulous attention to maintenance of organ perfusion, by means of the clinical measures described earlier, should ensure that respiratory arrest in association with epidural block occurs extremely seldom and that such an occurrence should be rapidly reversible, with proper management.
It has been claimed that extensive sensory blockade may result in loss of consciousness owing to lack of input to the reticular activating system. However, epidural block to T1 does not cause loss of consciousness. This requires complete afferent blockade, including blockade of cervical nerve roots and the cranial nerves (87).
Many factors may contribute to the respiratory effects of epidural block. At present, our knowledge in this area is meager. However, the documented changes produced by epidural block per se appear to be mild. For example, a sensory level of T3, associated with a motor level of T8, may be expected to result in essentially no change in vital capacity (VC) and functional residual capacity (FRC) in normal patients, so that respiration and the ability to cough are not impaired (118,119). In patients with severe pain, epidural block probably improves
VC and FRC as well as PaO2, at least in the early postoperative period (see also Chapter 39); this may result in improved respiratory exchange and more effective coughing (87,120,121,122,123).
VC and FRC as well as PaO2, at least in the early postoperative period (see also Chapter 39); this may result in improved respiratory exchange and more effective coughing (87,120,121,122,123).
Effect of Thoracic Epidural Anesthesia on Respiration
Since epidural anesthesia induces segmental block of spinal nerves, an adequate extension of the block of motor nerves can selectively affect respiratory muscles in the rib cage. Therefore the effect of TEA on the performance of the parasternal intercostal muscles was investigated by measuring electromyographic activity and length changes of the parasternal muscles in anesthetized, spontaneously breathing dogs (124). Thoracic epidural anesthesia caused rib cage distortion by impaired contraction of the parasternals and conceivably other respiratory muscles in the rib cage as well. Extrapolation to the clinical situation is difficult. However, in healthy awake volunteers, TEA caused a reduction of ventilatory response to CO2 during spontaneous respiration principally because of decreased contribution of the rib cage to tidal breathing (125). Mechanical impairment of rib cage movement can produce decreased ventilatory response to carbon dioxide, probably reflecting blockade of the efferent or afferent pathway (or both) of the intercostal nerve roots. These changes, however, are of an order unlikely to be of clinical relevance. Prevention of respiratory failure by blocking pain-related effects on ability to cough and breathe deeply usually outweighs any consideration of ventilatory impairment from the block itself, especially as diaphragmatic function may be improved.
Furthermore TEA does not impair the hypoxic drive; for example, the ventilatory response to progressive isocapnic hypoxemia as has been shown in non-premedicated patients (126). There appears to be no reason to avoid TEA in patients who are reliant on hypoxic drive, such as those with chronic airway disease.
Diaphragmatic dysfunction is a major determinant of the impaired respiratory function observed after upper abdominal and thoracic surgery. Mankikian et al. (1988) showed that TEA increased postoperative esophageal and gastric pressure and diaphragmatic motion indices of diaphragmatic function after upper abdominal surgery in humans, suggesting a partial reversal of the diaphragmatic dysfunction (127). These results, however, were based upon indirect measurements and may also reflect changes in abdominal muscle activity. Pansard et al. (1993) obtained direct diaphragmatic electromyogram recording from intramuscular electrodes that had been inserted into the costal and crural parts of the muscle during elective abdominal aorta surgery (128). The electrical diaphragmatic activity was increased but was not associated with improved diaphragmatic contractility. It seems unlikely that diaphragmatic activity increased during TEA as a compensation for reduction in parasternal muscle inspiratory activity produced by motor blockade, since rib cage motion did not change after thoracic epidural blockade. In an awake sheep model 24 hours after thoracotomy, there was a significant decrease of both costal and crural diaphragmatic shortening; following TEA using lidocaine, tidal volume increases were recorded, but there was markedly reduced rib cage expansion (129). This finding may be due to a shift of the work load of breathing from the chest wall to the diaphragm, thus explaining the unexpected observation that rib cage function decreased in this animal model. By using the same study design in humans undergoing thoracic surgery, this group observed a marked impairment of active diaphragmatic shortening, which was not reversed by TEA, despite improvement of other indices of respiratory function (130). This probably suggests that diaphragmatic contraction could not overcome the increased external forces placed upon it by other respiratory muscles. Differences in outcome of both investigations may be species-related (see also Chapter 7).
In summary, the effects of epidural anesthesia on diaphragmatic function after upper abdominal and thoracic surgery are complex. The most likely explanation for the TEA-related increase in diaphragmatic activity seems to be the interruption of an inhibitory reflex of phrenic nerve motor drive, either related to direct deafferentation of visceral sensory pathways or related to a diaphragmatic load reduction due to increased abdominal compliance.
Segmental high TEA can be used in patients with severe chronic obstructive pulmonary disease (COPD) and asthma undergoing chest wall surgery (131). In patients with bronchial hyperactivity, high TEA does not alter airway resistance, suggesting that reported cases of severe bronchospasm during epidural anesthesia are unrelated to sympathetic blockade and may be caused by mechanisms other than pulmonary sympathetic denervation (132).
Epidural Block and Motor Function
Clinical Applications of Deliberate Preservation of Motor Function
With respect to respiratory function, it is clear from the previous section that the aim is to use the appropriate drug and regimen to preserve motor function, and thus to permit deep breathing and coughing. In postoperative patients, continuous infusion of bupivacaine has proved to be an attractive method of achieving this goal. This method is described in detail in Chapter 43. Preservation of motor function also permits ultra-early ambulation, since patients who are pain-free are able to ambulate soon after surgery (87). It may be necessary to use vasopressors if epidural block is continued with only local anesthetic. Alternatives are to use dilute bupivacaine plus opioid (some risk of hypotension) or opioid alone (no risk of hypotension), as described in Chapter 43. Ultra-early ambulation probably decreases the risk of venous thrombosis and may decrease the hospitalization time (87). More controlled data are required (see also Chapter 7).
Clinical Applications of Motor Function Depression
In abdominal and hip surgery, depression of motor function is necessary during surgery. In this situation, the powerful motor blockade of etidocaine or lidocaine may be used (Fig. 11-7). Some of the motor effects are obtained by “deafferentation,” preventing reflex muscle contraction by blocking nociception before it reaches the spinal cord.
Factors determining motor effects of epidural block are as follows (see pharmacology section):
The local anesthetic drug: Ropivacaine has the least motor effects; etidocaine has the most potent effects.
Dose of drug: Degree of motor blockade is increased as dose of drug increases.
Repeated doses of drug: With “top-up” techniques, both motor and sensory blockade tend to become more intense with repeated doses; however, if dilute solutions of ropivacaine, levobupivacaine, or bupivacaine are used by
controlled continuous infusion, motor blockade can be kept to a minimum.
Epinephrine as an adjuvant increases the degree of motor blockade.
Epidural Blockade and Pregnancy
The known and potential physiologic effects of epidural block on mother, placenta, and fetus must be viewed in the light of contemporary knowledge of the physiology and pathophysiology of pregnancy, fetal physiology, and pharmacology. The detailed implications for regional anesthesia are discussed further in Chapter 24. By recognizing the modifications in management that are predicted by the impact of the physiologic changes of pregnancy, one can administer epidural anesthesia to gravid women with efficacy and safety.
Pharmacology of Epidural Blockade
The essence of the clinical pharmacology of epidural block is the provision of safe and effective neural blockade. To safely institute an epidural block, a knowledge of the physiology of epidural block is necessary, as well as a revision of the pharmacokinetics of local anesthetics as related to their administration by means of the epidural route. The efficacy of epidural block depends on this and on the clinical effects of the local anesthetics used (see Chapters 2,3,4,5).
In considering the pharmacokinetics of local anesthetics, both the systemic absorption and systemic disposition are of importance. Systemic absorption of local anesthetics limits the duration of nerve blocks and is of concern in view of systemic toxicity. The general absorption and disposition characteristics of local anesthetics are discussed in detail in Chapter 3. The potential for systemic toxicity should also be considered when choosing a local anesthetic agent for epidural use. The pharmacokinetic characteristics of the local anesthetics currently used for epidural analgesia and the implications for the time course of neural blockade and systemic toxicity have been reviewed in detail by Burm (133,134). Toxic effects of local anesthetics mainly involve the CNS and the cardiovascular system, depending on the rapidity of absorption from the epidural space and the total dose of the drug administered. This topic is covered in detail in Chapters 3, 4, and 5.
Thus, a thorough knowledge of the pharmacokinetics and toxicity of local anesthetics is a prerequisite to the safe use of epidural analgesia.
Sites of Action
Local anesthetics may act on the periphery of the spinal cord, the spinal roots, the dorsal root ganglia, and the extradural nerves (87). However, the spinal nerve roots, at the location where they leave the subarachnoid space and enter the nerve root sheath, are suggested to be the primary sites of action during spinal and epidural anaesthesia (87,135). This is substantiated by significantly higher tissue concentrations in the intradural spinal roots than in the spinal cord (136,137) and the close proximity of the epidural space and the nerve roots (135). Both dura and arachnoid-mater appear to be thinner in this region (138,139). In addition, the diffusion surface is increased by the dispersion of the bundles into individual fascicles (135). Subsequently, diffusion of local anesthetics from the epidural space through the dura mater into the CSF occurs to the periphery of the spinal cord (136,140).
Likewise, opioids rapidly gain access to CSF (see also Chapter 40) (141,142).
Likewise, opioids rapidly gain access to CSF (see also Chapter 40) (141,142).
Furthermore, extensions of the subarachnoid space provide a large area for penetration of the local anesthetic into the nerve structures and also possibly provide retaining pockets of high concentration of local anesthetics, limiting dilution into a greater pool of CSF (135). Despite lower tissue penetration of local anesthetics in the spinal cord (with concentration being highest in the lateral and posterior column and lowest in the gray matter) (137), involvement of the spinal cord in nerve blocking during epidural anesthesia has been demonstrated (143,144,145). Even lower tissue concentrations have been demonstrated in the dorsal root ganglia after epidural administration of local anesthetics (137). Nevertheless, this site has also been proposed as the primary site of action (138).
Longitudinal Spread of Solutions in the Epidural Space
The longitudinal spread of local anesthetics in the epidural space accounts primarily for the extent of epidural neural blockade, although they do not necessarily correspond exactly. This is because diffusion and vascular transport possibly influence the ultimate spread of analgesia (see Chapter 3). Longitudinal spread has shown to be more in a cephalad than caudad direction (146,147), and it depends largely on bulk flow during and after administration and on those structures in the epidural space that resist flow (148). In this context, the epidural space can be regarded as a reservoir that is collapsible, distensible, and leaky. The spread of analgesia may be modified by outflow of local anesthetics through the intervertebral foramina. Continuous positive airway pressure increased the spread of sensory blockade in TEA, primarily by a more caudad extension of sensory blockade (149).
Changes in the anatomy of the intervertebral foramina by disease or advancing age may alter the spread of analgesia by this mechanism (see Chapter 9).
Clinical Considerations for the Efficacy of Epidural Blockade
There is no question now that epidural block can be effective in nearly all cases if attention is paid to the anatomy, physiology, and pharmacology of the technique. Yet there are still many major medical centers throughout the world that hold the belief that epidural blockade has a high failure rate compared with subarachnoid blockade. This merely serves to underline the relatively recent acquisition of relevant data on which to base the effective use of epidural block.
Assessment of Epidural Blockade
In defining important factors in effective epidural block, the development of standardized methods of assessment of epidural block has been essential.
Sensory Block
Sensory block is graphed by testing for loss and return of pin-prick sensation (partial sensory block) in each dermatome on both sides of the body. An alternative method of testing initial onset is to use an alcohol swab to assess loss of temperature sensation, which is the most sensitive indicator of initial onset of sensory block (see Chapter 2, Table 2-1). Complete loss of touch sensation may also be charted (150,151).
From a “time-segment” graph can be obtained (a) time to initial onset and complete spread of analgesia, (b) time to regression of two segments and complete regression of analgesia, (c) total number of segments blocked on both sides of the body, (d) milliliters of local anesthetic per mean segmental spread (total segments R + L divided by two), and (e) area of the segment–time diagram (segment minutes), which can be related to the dose of local anesthetic, in segment minutes per dose. The latter expression is used to assess the development of tachyphylaxis (see later discussion).
Somatosensory Evoked Potentials
Cortical-derived somatosensory evoked potentials (SEPs) have been used in the qualitative assessment of the intensity of peripheral and central neural blockade (152,153,154,155,156,157,158).
Somatosensory evoked potentials reflect the net results of neuronal activities coming from peripheral nerves through the spinal cord to the brain. Somatosensory evoked potentials are generated by repetitive stimulation of peripheral nerves and can be monitored at several points along the sensory pathway, including over the spinal cord, subcortical structures, and cerebral cortex. Intraoperative monitoring of SEPs is an accepted technique to assess the functional integrity of the sensory pathways, particularly during spinal and scoliosis surgery (159).
Despite a clinically adequate block as assessed by pin-prick, afferent impulses generating SEPs still passed through an expected blocked area following epidural administration of bupivacaine, mepivacaine, or etidocaine. This probably indicates that total afferent blockade often is not obtained. Abolishment of SEP has only been accomplished using 1.5% etidocaine (154). This is possibly due to the ability of etidocaine to penetrate the white matter of the spinal cord more readily (144).
Sympathetic Block
Sympathetic block is assessed by measuring skin temperature with a telethermometer thermography, or temperature-sensitive papers. Alternatively, a digital plethysmogram may be used. Skin conductance can be measured in the clinical setting by use of the psychogalvanic response; reliable measurements are much more difficult than usually acknowledged. More precise, but of research application only, are the use of various sweat tests, such as cobalt blue and starch iodine, or the response of skin plethysmography to ice during venous occlusion plethysmography. A full discussion of the clinical and laboratory tests of sympathetic block is given in Chapter 39.
Motor Block
Motor block is usually assessed by use of the Bromage scale for motor blockade in the lower limbs (87) (Table 11-5).
Table 11-5 Bromage scale | ||||||||
---|---|---|---|---|---|---|---|---|
|
Motor blockade in the lower limbs can be assessed with reference to specific myotomes (e.g., L2, hip flexion) (151). A score of 0 is assigned for no block and 1 for complete block (no movement) at each joint on each side. Thus, maximal motor block is present bilaterally with a score of 10:
Right | Left | |
---|---|---|
Hip flexion (L2) | 1 | 1 |
Knee extension (L3) | 1 | 1 |
Ankle dorsiflexion (L4) | 1 | 1 |
Great toe dorsiflexion (L5) | 1 | 1 |
Ankle plantar flexion (S1) | 1 | 1 |
5 + 5 = 10 (complete motor block) |
This test removes some observer error because only a “move” (0) or “no move” (1) decision needs to be made at each joint.
An onset profile for motor blockade can be presented as a “myotome score–time” diagram. For research purposes, Axelsson reported an apparatus that measures maximal isometric strength by a force transducer at ankle, knee, and hip. This provides objective, reproducible measurements of muscle power (160).
Abdominal muscle power may be assessed by the rectus abdominis muscle (RAM) test (Table 11-6) (161). This is useful in abdominal surgery, when abdominal muscle blockade is required rather than lower limb muscle blockade. On the other hand, the Bromage scale is useful for lower limb surgery. Both scales may be used when a comprehensive picture is required: RAM-test (T5–T12) and Bromage scale (L1–S2).
Testing of 100% and 80% power has limitations in patients with vasodilation; blood pressure and pulse rate must be carefully monitored if these tests are to be used.
A broad comparison of agents used for epidural block can be compiled based on their success rate in producing motor and sensory block. Because different methods of testing have been used in many studies, the comparisons are only qualitative (Fig. 11-7).
Electromyography
Few studies have used the more quantitative method of electromyography (EMG), although this would provide more sensitive assessment.
Reflex Response
Under general anesthesia without muscle relaxation, sensation can still be crudely assessed by use of reflex response to pinch by a forceps at appropriate segmental levels. Alternatively, the tendon reflexes in the lower limbs give a gross index of both motor and sensory block, while reflexes such as those of the cremaster, anal, and abdominal muscles may also be useful as a gross guide to adequacy of blockade.
Factors Affecting Epidural Blockade
Many factors may affect the efficacy, spread of blockade, fiber types blocked, and other aspects of epidural blockade: site of injection and nerve root size; age; position; speed of injection; dose and choice of local anesthetic; adjuvants; number and frequency of injections.
Table 11-6 RAM Test of Abdominal Muscles | ||||||||||
---|---|---|---|---|---|---|---|---|---|---|
|
Site of Injection and Nerve Root Size
Blockade tends to be most intense and has the most rapid onset close to the site of injection. The subsequent spread of analgesia depends to some extent on whether the injection is made in thoracic or lumbar regions.
After lumbar epidural injection, a somewhat greater cranial than caudal spread of analgesia occurs and there may be a delay in the L5 and S1 segments. The delay in onset at these segments appears to be due to the large size of these nerve roots (162).
After midthoracic epidural injection, analgesia spreads quite evenly from the site of injection. However, the upper thoracic and lower cervical segments are resistant to blockade because of the large size of the nerve roots and the large number of nerve fibers within them. Repeated doses by the mid thoracic route eventually may cause analgesia to spread into lumbar and sacral segments, with the expected lag in onset at L5–S1. Careful control of dose in the thoracic region permits sparing of the lumbar segments and thus avoidance of sympathetic block in the lower limbs and maintenance of normal bladder function—that is, a true segmental block. Similarly, a small dose injected at L2–L3 for labor pain may block only T11 and L3–L4 segments, while it spares the sacral segments.
The profile of onset of caudal epidural block spreads upward from S5, and the S1 segment is the last to be blocked, as expected.
Age
Over the past several years, the anesthesiologist has been faced with a growing number of elderly patients presenting for surgery. Epidural anesthesia has enjoyed a resurgence of popularity for elderly patients undergoing surgery in areas amenable to conduction anesthesia. With advancing age, anatomic changes do occur in the epidural space (136). In the young individual, the areolar tissue around the intervertebral foramina is soft and loose. In the elderly, this areolar tissue becomes dense and firm, partially sealing the intervertebral foramina (87). With aging, the dura becomes more permeable to local anesthetic because of significant increase in the size of the arachnoid villi (138). Discrepancies exist among studies in which the influence of age on epidural anesthesia has been assessed. The classic study of Bromage regarding the influence of age on epidural spread reported a strong relationship between age and the epidural segmental dose requirement (ESDR); that is, the amount (dose) of local anesthetic required to block one spinal segment (163). Assuming a linear dose-relationship, Bromage demonstrated that, with age, the ESDR decreased in a linear way. In contrast to Bromage’s assumption, others have found no direct linear relationship between volume and anesthetic spread (164,165,166). The greater the total amount used, the greater the ESDR calculated. From this it can be concluded that the results of Bromage’s study concerning the linear decrease of ESDR with age are questionable because he assumed a direct linear relationship between the amount (dose) of local anesthetic and extent of anesthesia and because he used variable total anesthetic amounts (163). Using a given dose (fixed volume and concentration), other investigators have found a significantly greater number of spinal segments blocked in older patients. However, the magnitude of this effect was small: only one to three segments more in the elderly patients compared to younger adult patients (Fig. 11-8) (164,165,167,168,169,170,171). When using different volumes, the dose-effect relationships varied with these volumes (166,172).
The spread was also greater in the elderly patients than in younger ones after epidural anesthesia with the relatively new long-acting local anesthetics ropivacaine (173) and levobupivacaine (174). The higher spread with ropivacaine in elderly patients was accompanied with a high incidence of hypotension and bradycardia (Fig. 11-9). This problem is a particularly important issue in elderly patients with cardiovascular disease such as hypertension, because the risk for ischemia secondary to hypotension is increased (175,176).
Since aging is associated with reduced β-adrenergic responsiveness, a small dose of epinephrine added to a local anesthetic does not appear to be a reliable detector of unintentional intravascular injection of local anesthetic solution (177).
Age has also been shown to be associated with a higher upper level of analgesia following TEA of a fixed dose; increased levels of analgesia with increasing age have been attributed to reduced leakage of local anesthetic solution because of progressive sclerotic closure of intervertebral foramina (87,136). Also, the cephalad spread of radioactivity after epidural injection of 131I mixed in 2% lidocaine was higher in patients older than 50 years than in those younger (147). Radiologic studies, however, have failed to show a relationship between age and spread in the epidural space (146). It is possible that radiopaque material and local anesthetic do not spread in an identical manner. On the other hand, the increased permeability of the dura with aging, as described by Shantha and Evans (138), may contribute to the higher levels of analgesic spread in the elderly. Usubiaga reported that older patients have a higher residual pressure and that a positive relationship exists between residual epidural pressure and the extent of analgesic spread. Also, increased epidural compliance and decreased epidural resistance with advancing age may contribute to this enhanced spread in the elderly (178).
The onset time to maximal caudad spread has been reported to decrease with advancing age following epidural administration of bupivacaine, thus allowing surgery in areas innervated
by these segments to be started sooner in older patients than in younger (170,171). In addition, a more rapid onset and enhanced intensity of motor blockade has been shown in older patients (171). With aging, the neural population declines steadily within the spinal cord, and peripheral nerves show a linear reduction in conduction velocity, especially in motor nerves (179). This could make older patients more sensitive to local anesthetics, which is probably (partially) the cause of the shorter onset time of analgesia in the caudad segments and the altered motor block profile. Nydahl (167) reported a shorter duration of motor blockade, as assessed by EMG recordings, following epidural administration of epinephrine-containing bupivacaine solutions. This may be attributed to a stronger reaction in younger subjects to epinephrine (180).
by these segments to be started sooner in older patients than in younger (170,171). In addition, a more rapid onset and enhanced intensity of motor blockade has been shown in older patients (171). With aging, the neural population declines steadily within the spinal cord, and peripheral nerves show a linear reduction in conduction velocity, especially in motor nerves (179). This could make older patients more sensitive to local anesthetics, which is probably (partially) the cause of the shorter onset time of analgesia in the caudad segments and the altered motor block profile. Nydahl (167) reported a shorter duration of motor blockade, as assessed by EMG recordings, following epidural administration of epinephrine-containing bupivacaine solutions. This may be attributed to a stronger reaction in younger subjects to epinephrine (180).
Epidural anesthesia carries some problems in elderly patients. The technique is technically more difficult, and so a chance of a failure is always present. This is partially attributed to the fact that the ligamentum flavum probably changes into a form that is easily ossified (181). The more extensive spread of analgesia is likely to be accompanied by more extensive sympathetic blockade. Therefore, prevention of hypotension by intravenous (IV) administration of crystalloid fluids will be important in older patients. It should be emphasized, however, that rapid volume preloading constitutes a potential risk in elderly patients with poor cardiac function, in whom there is a risk of pulmonary edema and cardiac failure.
With epidural anesthesia, a decline in the thermoregulatory response occurs with age, as has been shown by the decrease in core temperature (182). Consequently, the postoperative rewarming process will occur more slowly in older patients. Lumbar epidural anesthesia (LEA) using lidocaine did not affect the resting ventilation parameters, such as minute ventilation and tidal volume, in older patients, and it stimulated the ventilatory response to hypercapnia to the same degree as in young patients (126). Therefore, LEA appears to be a safe technique in elderly patients.
Important considerations for the use of epidural anesthesia in pediatric patients is covered in Chapter 27.
Position
Comparison of sitting and lateral positions for epidural block reveals no significant differences in cephalad spread (183). An exception is the obese patient, who achieves a lower level of block when seated (184). Caudad spread of block in seated patients is slightly favored by the sitting position (185). From these studies. it seems that the differences between sitting and lateral positions are small. The lateral position favors spread of analgesia to the dependent side in both pregnant and nonpregnant patients (186,187,188), but the differences are small. In surgical patients, however, Seow and associates found that onset of sensory and motor block was significantly more rapid on the dependent side and had a longer duration. In addition to being more rapid in onset, motor blockade was greater on the dependent side at all time intervals tested out to 40 minutes after injection. Onset time for sympathetic block was not faster on the dependent side, but duration of maximum elevation of skin temperature was greater on the dependent side (188). The differences in sensory and motor block are great enough to indicate an advantage in placing patients on the operative side during epidural block before lower limb surgery (188). There appears to be no correlation between spread of analgesia and weight and height in adults (87).
Speed of Injection
Increasing the speed of injection has no effect on the bulk flow of solutions in the epidural space (146,147). Also, spread of analgesia is only minimally influenced. However, rapid injection of large volumes of solution may increase CSF pressure (189), decrease spinal cord blood flow, increase intracranial pressure, and pose a risk of spinal or cerebral complications. In susceptible patients, sudden increases in CSF pressure may compromise spinal cord blood flow (see Chapter 12), and this may increase susceptibility to neurotoxicity (189) or, in patients with atherosclerosis, may possibly cause “spinal stroke.” Evidence exists that nutritive vessels crossing the perineurium of nerves are subject to a pathologic “valve” mechanism initiated by perineurial edema (190). This edema could be initiated by sudden increases in CSF pressure. Subsequent hypotension owing to sympathetic block may decrease spinal cord flow if CSF pressure and perineurial pressure remain high. A combination of these effects could result in neural damage (see also Chapter 12).
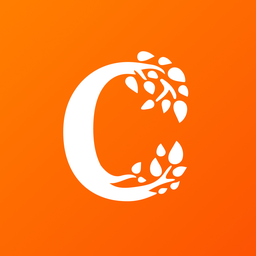
Full access? Get Clinical Tree
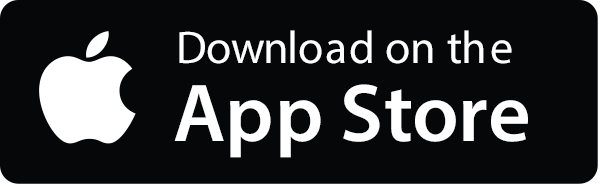
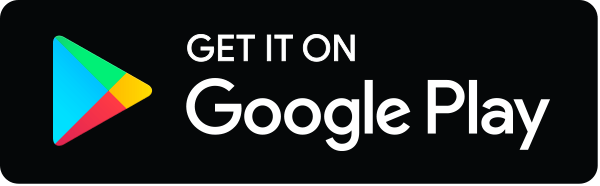
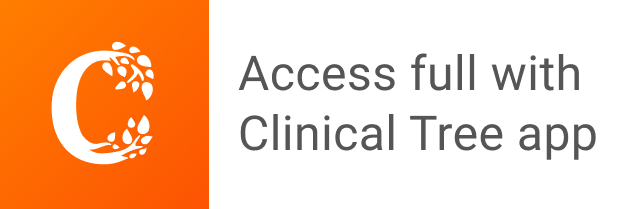