Advances in echocardiography have been responsible for enhancing our understanding of the mechanisms and progression of cardiovascular diseases. The introduction of new echocardiographic imaging modalities has greatly aided our ability to obtain early and precise disease diagnosis, while simultaneously allowing better means to guide medical or surgical care for patients with heart diseases. In this chapter, we discuss the role of these emerging echocardiographic technologies in the perioperative period.
Three-dimensional (3D) echocardiography has a long history with the first 3D-reconstruction of two-dimensional (2D) images described in 1974 and the first human 3D–transesophageal echocardiogram (TEE) performed in 1992.1,2 Until recently, 3D-TEE was performed using a rotational approach for sequential data acquisition, gated to electrocardiography (ECG) and respiration.2,3 Volume rendering and cropping of these reconstructed 3D volume data sets allowed display of structures of interest. However, these earlier approaches were limited by time-consuming acquisition and reconstruction processes (15 to 30 minutes), frequent artifacts, and the need for offline processing.4,5 As a consequence, reconstructive 3D-TEE remained primarily a research tool and did not make it into routine clinical practice. With the recent introduction of a 3D fully sampled matrix array TEE transducer, many of these limitations have been overcome. Based on novel electronic circuitry and a matrix array design of piezoelectric crystals within an otherwise conventional TEE probe, this new technology allows both real-time acquisition as well as live display of 3D images. This real-time 3D-TEE (RT-3D-TEE) system allows for excellent visualization of the mitral valve (MV), the interatrial septum (IAS), the left atrial appendage (LAA), pulmonary veins, and the left ventricle (LV), while imaging of the aortic valve (AV) and tricuspid valve (TV) will require further technological improvements.6,7 Due to its unique features of real-time acquisition, online rendering and cropping capabilities, accurate identification of the precise pathology and location of cardiac disease, and prompt quantification of 3D structures with built-in software, RT-3D-TEE is expected to soon become a standard of perioperative care and clinical practice. However, this emerging technology requires the echocardiographer to acquire new sets of skills to acquire and manipulate the 3D data sets so that they can reveal valuable information. With an emphasis on RT-3D-TEE, the following sections will combine practical recommendations with some of the key features of the emerging 3D technology.
Technologic advances in the 1990s have allowed improved reconstructive 3D echocardiography based on the acquisition of multiple, gated image planes using ECG and respiratory gating that limits the amount of motion artifacts. Post-processing of acquired images results in further optimization of these reconstructive 3D images. One significant drawback of these approaches to 3D echocardiography was that live, real-time (RT) imaging could not be achieved because the different imaging planes are acquired sequentially. Further, sequential and gated acquisition frequently resulted in motion artifacts. In the late 1980s, a sparse array matrix transducer containing 256 elements was designed to develop a new approach to 3D echocardiography and to overcome some of these issues. While this transducer generated different cut-planes from a 3D volume online, it ultimately displayed RT rendered 3D images.8–10 Further advances in crystal and computer technology allowed for the introduction of matrix-array transducers for use in transthoracic echocardiography (TTE). Matrix-array transducers utilize a greater number of imaging elements (>2500), which are capable of generating RT rendered 3D images.11,12 More recently, the reduction in the size of the transducer footprint along with improved ultrasound crystal technology led to the introduction of the RT-3D-TEE transducers and real-time capable ultrasound systems.
The first and currently only clinically available RT-3D-TEE transducer is the X7-2t TEE (Philips Medical Systems, Andover, MA, USA) transducer, which combines xMATRIX technology and PureWave crystal technology (Figure 24–1). PureWave crystal technology represents a new class of piezoelectric crystals with remarkably enhanced electromechanical properties. With the power of 150 computer boards, xMATRIX technology utilizes 2500 fully sampled elements for 360° focusing and steering. This cutting-edge technology creates two live full-resolution planes created simultaneously (xPlane imaging) and, therefore, enables the parallel acquisition of diagnostic data without changing the scan angle (Figure 24–2). Further, the system enables live or RT volume imaging over a single heartbeat with unlimited planes in all directions, allowing the acquisition and rendering of true RT-3D full volume data. Gated images are acquired over multiple heartbeats and are displayed as a loop generated by stitching together subvolumes over consecutive cardiac cycles. Similar to other ultrasound technologies, RT-3D-TEE imaging obeys the laws of physics and is limited by frame rate, image resolution, and sector size.13 Importantly, RT-3D systems provide all conventional modalities such as 2D multiplane imaging, M-mode, pulsed- and continuous-wave Doppler, as well as color Doppler imaging. In addition, the system offers the following four 3D imaging modes:
- Live 3D: This real-time mode displays a fixed pyramidal data set of approximately 50° × 30° by the depth of the initial 2D image that conveniently can be used to visualize any cardiac structure located in the near field (Figure 24–3). Movement of the TEE probe will result in a live (real-time) change of the 3D image. Live 3D allows for quick 3D imaging and immediate return to a 2D mode. Using live 3D, a thick slice (90° × 1°) representing an enhanced 2D image may also be displayed and rotated in space.
- 3D Zoom: This displays a truncated but magnified pyramidal data set of variable size (pyramidal dimensions vary from 20° × 20° up to 90° × 90°). Upon activation, the 3D-zoom mode displays a biplane preview screen showing the original view and the correspondent orthogonal image. Careful placement and sizing of the zoom sector over the region of interest with minimized sector-width to improve temporal resolution are important to optimize image quality and frame rate (Figure 24–4).
- Full Volume: This mode provides a pyramidal data set (approximately 65° × 60° up to 100° × 100°), which allows the inclusion of a larger cardiac volume at frame rates greater than 30 Hz. The wide-angle data set is compiled by merging four to seven narrower RT-3D pyramidal wedges or subvolumes obtained over four to seven heartbeats. To minimize artifacts in the anesthetized patient, full volume loops should be acquired when ventilation is held and electrocautery is not used. With the goal of minimizing artifacts in the intraoperative setting, it is recommended that full volume loops be acquired at the beginning of the comprehensive TEE exam, before the start of surgery. Artifacts cannot be avoided in patients with arrhythmias, and stitch artifacts delineate subvolumes and may make the interpretation of imaged structures impossible (Figure 24–5). A full volume loop of the LV is acquired based on the 2D midesophageal four-chamber view. Once the full volume mode is activated, a biplane image with the four-chamber view and the correspondent orthogonal plane is displayed on the screen. The first obtained 3D volume is displayed as an automatically cropped image showing 50% of the volume and mirroring the midesophageal four-chamber view (Figure 24–6A). Resetting the crop plane allows display of the entire pyramidal data set (Figure 24–6B). The full volume can be further processed offline by rotating and cropping to visualize specific structures inside the pyramid. Cropping can be performed either by using one of six available cropping planes selected from a 3D cropping box or by using a freely adjustable plane. Acquired full volumes can also be used for volumetric quantification of the LV using available built-in software (QLAB, Philips Medical Systems, Andover, MA, USA, version 6.0).
- 3D Color Full Volume: Similar to the acquisition of a full volume, the wide-angle color data set is compiled by merging 7 to 14 narrower RT-3D pyramidal wedges and is similarly prone to artifacts introduced by arrhythmias, movement, or electrocautery (Figure 24–7). For this mode, it is important to place the area of interest (eg, the regurgitant jet) in the center of the sector. The remainder of the acquisition is identical to that used for full volume acquisition. Due to the large amount of data incorporated in 3D color Doppler full volume data sets, the resulting pyramidal volumes do not exceed 60° × 60° and have a limited frame rate (<20 Hz).
Figure 24-2.

Bioprosthetic mitral valve as imaged with the xPlane mode (biplane mode). This mode displays two full-resolution planes created simultaneously and therefore enables the parallel acquisition of diagnostic data without changing the scan angle. The scan angle of the left plane (10°) is selected before initiating xPlane imaging; the scan angle of the right plane (100°) can be freely adjusted while imaging.
Figure 24-4.

Three-dimensional TEE zoom en face view of a normal mitral valve with the anterior mitral leaflet (AML) on the top and the posterior mitral leaflet (PML) on the bottom. The orientation of the image is similar to the surgeon’s view. (AV, aortic valve; ALC, anterolateral commissure; PMC, posteromedial commissure.)
Figure 24-5.

A 3D-TEE full volume data set is compiled by merging four to seven narrower 3D pyramidal wedges or subvolumes obtained over four to seven undisturbed heartbeats. The enface image in (A) demonstrates stitch artifacts (demarcation lines) that delineate subvolumes and may make the interpretation of the imaged structures impossible. (B) delineates the subvolumes in a cropped version of the same full volume data set.
Approximate measurements on acquired images can be performed easily by using the 3D grid with a specified dot-to-dot distance for estimating dimensions of cardiac structures such as the mitral valve annulus. More sophisticated and accurate measurements require the use of built-in software (QLAB). This software contains several programs including the Mitral Valve Quantification (MVQ), the 3D Quantification Advanced (3DQAV), and the simpler 3D Quantification (3DQ). MVQ and 3DQAV are described in detail below. 3DQ allows simple quantitative assessment of any 3D data set (eg, area, distance).
Acquired 3D images should always be rotated and orientated to display a specific anatomic or surgical perspective. Cropping (or slicing) of the 3D image along all three axes (X, Y, and Z) is made possible along six orthogonal planes or based on multiplanar reconstruction planes (Figure 24–8A). In addition, a freely adjustable cropping plane allows more sophisticated cropping in alignment to any cardiac structure of interest (Figure 24–8B). Simply touching the reset-cropping button can reverse any cropping and will restore the original 3D image.
Figure 24-8.

Full volume data set (A) of the left ventricle displayed in three multiplanar reconstruction planes (green, four-chamber view; red, two-chamber view; and blue, short-axis view) along with the 3D image displayed in a slice plane fashion (right lower quadrant). Using a cropping box tool, cropping (or slicing) of the 3D image can be achieved along six orthogonal planes. (B) illustrates alternative cropping using a freely adjustable cropping plane that allows more sophisticated cropping in alignment with any cardiac structure of interest. The image shows the purple cropping plane placed over a bilobed left atrial appendage.
The mitral valve (MV), with its complex saddle-shaped configuration, presents one of the most challenging structures to be assessed with 2D-TEE. Two-dimensional TEE imaging of the MV requires a mental integration of several views for accurate assessment and is, therefore, dependent on observer experience and expertise. In addition to its complex anatomic structure, the interrelationship of the MV to chordae, papillary muscles, and myocardial walls makes it particularly suited to 3D assessment. In this context, 3D echocardiography allows a more accurate identification of the etiology and mechanism of MR and is more sensitive than 2D echocardiography in identifying the location of the pathology leading to MR, especially in patients with bileaflet and commissural defects.4,14–16 In addition, the severity of MR can be more accurately determined using 3D color Doppler echocardiography,17 and specific geometric shapes for different MR pathologies can be identified.18–20
With 3D-TEE, the effective mitral valve area (MVA) may be assessed by carefully cropping through the MV annular plane. In this context, studies have confirmed that 3D echocardiography provides a more accurate measurement of the MVA compared to standard 2D-based measurements and shows the best agreement with invasive methods.21–23 Importantly, the 3D measurements had the additional advantage of being associated with lower intraobserver and interobserver variability. Further, 3D echocardiography has been used for guidance during percutaneous mitral valvuloplasty and has been shown to be a suitable technique for monitoring its efficacy and complications.23–25 This technique has become the procedure of choice in patients who are considered at high surgical risk, eg, pregnant women;26,27 however, the need for surgery after MV valvuloplasty is not uncommon.28 RT-3D-TEE might become a gold standard in describing the morphology of commissures, which in turn predicts outcome after percutaneous balloon mitral valvuloplasty.29
RT-3D-TEE provides superb visualization of prosthetic MVs and annuloplasty rings (Figure 24–9);6,30 thus, it might help in identifying the location of a paravalvular leak.31 Three-dimensional TEE has been shown to provide complimentary information in patients with an Alfieri stitch and may aid in long-term follow-up (Figure 24–10).32 Three-dimensional TEE provides additional information in patients with a postoperative MV dehiscence, and thus may help in planning an optimal surgical intervention.30 Prosthetic valve endocarditis remains a challenging diagnosis, especially for TEE. However, initial experiences suggest that 3D-TTE might improve the sensitivity of detecting endocarditis.33
Figure 24-9.

En face view of a mechanical double tilting disc MV prosthesis (A). A stented bioprosthetic MV is displayed in (B) as viewed from the left ventricular side. (C) represents a 2D-TEE image of a mechanical double tilting disc MV prosthesis. Imaged with the multiplane angle at 90°, this image reveals the significant anterior dehiscence of this MV prosthesis that is easily recognized in the RT-3D-TEE en face view displayed in (D).
It is expected that RT-3D-TEE will soon be integrated into routine perioperative practice in patients with MV pathologies. Its unique ability of real-time acquisition, online rendering and cropping capabilities, and accurate identification of the precise location and pathology leading to MV disease, together with its ability to define the severity of mitral regurgitation/mitral stenosis (MR/MS) by direct assessment of effective regurgitant orifice and volume, even for asymmetrically shaped regurgitant jets, will likely help to transition this modality into standard of care.
Imaging Techniques. With RT-3D-TEE, a comprehensive assessment of the MV involves the acquisition of an en face view, a full volume image, and a 3D color full volume image. The en face view mirrors the surgical view from the left atrium down to the MV. This view is routinely generated using the 3D-zoom mode based on the midesophageal four-chamber view, and by rotating the obtained image to display the aortic valve at the 12 o’clock position as the midpoint of the anterior annulus. Depending on the frame rate and line density settings, this commonly results in superior-quality volume-rendered images of the anterior leaflet at the top and the posterior leaflet at the bottom of the image (see Figure 24–4). Three-dimensional zoom MV images may then be manipulated such that the MV may be viewed from either atrial or ventricular perspectives, which is another unique feature of 3D imaging. The full volume data set allows assessment of the interrelationship between the MV, the papillary muscles, the myocardial walls, and the left ventricular outflow tract. Using 3D color, the size and geometry of regurgitant jets can be visualized and the exact quantification of effective regurgitant orifice areas (EROAs) can be obtained (Figure 24–11). These images can be supplemented by 3D quantitative assessment of the MV using built-in software (MVQ, QLAB). The MV Quantification (MVQ) feature offers a semiautomated analysis package for accurate modeling of the mitral annulus, valve commissures, leaflet coaptation, leaflet topography, aortic orifice to mitral valve angle, etc. (Figure 24–12). Reconstructive approaches to assess the MV with 3D-TEE (Siemens, Mountainview, CA, USA) paired with online computer software (TomTec Imaging Systems GmbH, Munich, Germany) allow for similar quantification of the MV.34
Figure 24-11.

Three-dimensional TEE color full volume of the MV displayed in three multiplanar reconstruction planes (green, red, and blue MPRs) along with the 3D image displayed as an en face view of the MV (right lower quadrant). The left lower quadrant (blue MPR) depicts an elliptical-shaped vena contracta (VC) as often seen in functional mitral regurgitation. This was achieved by carefully cutting the MV at the annular plane.
Figure 24-12.

(A) through (F) display the RT-3D-TEE views as well as the corresponding digital pictures of the surgical view of a mitral valve (MV) and 3D reconstructions (MV model) in a patient with a large P2 prolapse, ruptured chords, and severe mitral regurgitation. (A) shows the 3D zoom en face view of this MV, (B) the corresponding digital picture taken by the surgeon, (C) the 3D zoom en face view of the repaired MV (ring annuloplasty, Goretex chords, and Alfieri stitch), and (D) shows the digital picture taken by the surgeon following repair. Finally, (E) demonstrates the 3D quantitative assessment of the MV using built-in software (MVQ, QLAB) before the repair and following repair (F). The MV quantification software offers a semiautomated analysis package for accurate modeling of the mitral annulus, valve commissures, leaflet coaptation, and leaflet topography. (Al, anterolateral commissure; PM, posteromedial commissure)
The evaluation of global and regional left ventricular (LV) function is an essential part of a routine perioperative TEE examination. To date, the assessment of the LV ejection fraction (EF) is mainly performed by “eye-balling,” which relies on the echocardiographer’s experience and ability to visually integrate spatial information. Further limitations of 2D-TEE assessment of the LV EF are attributed to the use of foreshortened views of the LV and the reliance on geometric assumptions to calculate volumetric parameters. The addition of a third dimension is expected to overcome some of these limitations, especially in patients with cardiomyopathies or wall motion abnormalities where the geometric assumptions may lead to incorrect estimations of LV function. In this context, 3D echocardiography along with built-in quantification software that is based on semiautomated endocardial border detection allows the echocardiographer to obtain fast and accurate measurements of global and regional LV function.35–37 Studies comparing magnetic resonance imaging (MRI) with 3D echocardiography for the assessment of LV mass and function show very good correlation and agreement that is superior to 2D echocardiography.38 This also holds true for RT-3D-TTE assessment of patients with cardiomyopathies or regional wall motion abnormalities secondary to myocardial infarction, where LV geometry is frequently abnormal.39–41 A recent study suggests that LV function assessment based on 3D-TEE data offers more reliable perioperative quantification, especially for less experienced users.42 However, further research comparing 3D-TEE to a gold standard such as MRI is required to confirm that 3D-TEE is superior to 2D-TEE in assessing LV function.
Imaging Techniques. The best mode to assess global and regional LV function by 3D-TEE is the full volume mode, which is acquired from the midesophageal four-chamber view. Using built-in software, the 3D Quantification Advanced (3DQAV) program, data for both global LV function as well as regional wall motion abnormalities are obtained in a semiautomated fashion. The system relies on automatic endocardial border detection and border tracking algorithms, which can be edited manually. The first step after acquisition is a manual definition of the septal, lateral, anterior, inferior, and apical endocardial border of the LV in the end-systolic and the end-diastolic frames, followed by activation of an automatic border-tracking algorithm (Figure 24–13A). The system will then calculate end-systolic as well as the end-diastolic volumes by summation of the voxels enclosed by the endocardial borders. Thereafter, global stroke volume and EF are derived. The obtained “shell view” is subdivided into 17 regions, which are analyzed separately by performing the “segment analysis,” and 17 segmental time-volume waveforms are displayed simultaneously, offering the possibility of more objective wall motion comparisons (Figure 24–13B). Activation of the “show reference mesh” feature within the software displays the end-diastolic surface mesh as a diastolic reference point (Figure 24–13D). Other viewing modes include the “iSlice” view, which displays up to 16 simultaneously moving short-axis views of the LV and allows verification of appropriate endocardial border detection, as well as the “Slice Plane” view, which shows a moving LV surface mesh within three orthogonal axis planes (Figure 24–13C).
Figure 24-13.

(A) shows a 3D-TEE full volume of the entire heart displayed in three multiplanar reconstruction planes (MPRs) (green, four-chamber view; red, two-chamber view; and blue, midpapillary short-axis view) along with the 3D image displayed as a slice plane view demonstrating the heart within these three MPRs (right lower quadrant). Manual definition of the septal, lateral, anterior, inferior, and apical endocardial border of the LV in end-systole and end-diastole, followed by an automatic border-tracking algorithm and segmental analysis, will display the LV shell in 17 segments (B) along with the corresponding segmental time-volume waveforms. The slice plane view (C) and the shell view (D) with an end-diastolic reference mesh are alternative options for display of the data.
Three-dimensional assessment of the native AV and TV is more difficult when compared to 3D imaging of the MV. Both valves can be optimally visualized only in 18% (AV) and 11% (TV) when using RT-3D-TEE, largely because these valves are anterior structures with a longer distance from the transducer, are associated with a less favorable angle of insonation, and have thinner leaflets compared to the MV.6
Imaging Techniques. The best mode to assess the AV appears to be the Live 3D mode (see Figure 24–3), while the TV is best assessed using the 3D-zoom or full volume mode. Occasionally, the 3D full volume mode might offer more detailed information because of higher frame rates. This mode will also allow assessment of both atrioventricular valves and the AV simultaneously. Thickening and calcification of the AV cusps mostly facilitates RT-3D-TEE imaging of the AV, but significant calcification results in similar drop-out (shadowing) as seen with 2D-TEE. Cropping of a 3D-TEE image assists in the planimetric assessment of the AV area. RT-3D-TEE may also be of benefit in differentiating bicuspid from tricuspid anatomy (Figure 24–14A), assessing involvement of the AV or coronary arteries during acute dissections (Figure 24–14B) of the ascending aorta, or in diagnosing AV endocarditis.
Figure 24-14.

(A) shows a calcified bicuspid aortic valve (AV) imaged with live 3D in an open position. (B) displays a dissection of the ascending aorta originating very close to the AV. (C) displays the en face view of a normal tricuspid valve with a pulmonary artery catheter in place acquired using 3D zoom. (D) demonstrates a 3D zoom of the left atrial appendage adjacent to the ligamentum of Marshall, the left upper pulmonary vein, and the mitral valve. (LA, left atrium; NCC, noncoronary cusp; RCC, right coronary cusp; LCC, left coronary cusp; P, posterior leaflet; S, septal leaflet; A, anterior leaflet; PAC, pulmonary artery catheter; LUPV, left upper pulmonary vein; LOM, ligament of Marshall; LAA, left atrial appendage; MV, mitral valve.)
Optimal visualization of the TV using RT-3D-TEE is achieved only in a small percentage of patients (Figure 24–14C).6 It remains to be seen if RT-3D-TEE will improve the accuracy in the assessment of TV dysfunction similar to what has been described for the MV. Early reports using 3D-TTE suggest that assessment of tricuspid regurgitation is feasible in most patients and that the shape of the vena contracta is more ovoid than that of mitral regurgitant jets.43
Three-dimensional echocardiographic imaging of the pulmonic valve is even more difficult. This is due to the fact that the pulmonic valve is located most anteriorly and its cusps are the thinnest of all cardiac valves. The use of RT-3D-TTE suggests that pulmonic insufficiency may be assessed more accurately and with a lower intra- and interobserver variability when compared with 2D imaging.44 To date, no studies have assessed the utility of RT-3D-TEE for the assessment of pulmonic stenosis or insufficiency.
TEE is considered the gold standard imaging modality for detection of left atrial appendage (LAA) thrombi. Despite a relatively high specificity, 2D-TEE imaging may overestimate the incidence of thrombi partly due to the complex 3D morphology of multi-lobed appendages.45 The complex structure of the LAA lends itself well to 3D assessment. Several case reports using RT-3D-TTE or reconstruction 3D-TEE showed that 3D assessment enables excellent visualization of the LAA anatomy and function (see Figure 24–8B).46,47 Further, RT-3D-TEE provides excellent visualization of the LAA orifice, which may optimize the guidance for the placement of LAA occlusion devices.48 Successful application of RT-3D-TEE to confirm stable catheter position along the entire length of the ligament of Marshall during left atrial catheter ablation for atrial fibrillation has also been described. RT-3D-TEE could potentially enhance lesion delivery during left atrial catheter ablation for atrial fibrillation to improve efficacy and safety.49 Further, RT-3D-TEE might become the method of choice to more accurately assess the LAA, especially to distinguish thrombi from anatomical variants, and might alter the course of therapy in patients with atrial fibrillation, particularly during placement of an LAA occlusion device.50
Imaging Techniques. The LAA can be best visualized by using the 3D-zoom mode obtaining the en face view of the LAA with the adjacent ligament of Marshall (see Figure 24–14D).
Although the introduction of RT-3D-TEE to the operating room has truly added a new dimension to perioperative imaging, a number of significant limitations remain. First, while 3D zoom and Live 3D present truly real-time modes, the acquisition of a 3D full volume as well as a 3D color full volume are based on automatic reconstruction from multiple subvolumes, and, therefore, are prone to artifacts resulting from arrhythmias, ventilation, and other movements. Second, as RT-3D-TEE obeys the same physical laws as 2D ultrasound, poor 2D image quality will likely translate into similarly poor 3D image quality that adds little to no value to the overall assessment. Third, image acquisition of 3D full volumes and 3D color full volumes and associated quantification remain time-consuming. Fourth, although built-in software offers a truly novel and promising approach to quantitative assessment of 3D data sets of the MV and LV, this assessment is static, and dynamic changes during the cardiac cycle are not reflected. Finally, as RT-3D-TEE technology represents a brand-new technology, its use will naturally prolong a comprehensive TEE examination. This is especially true when more sophisticated quantification using built-in software is performed.
Ultrasound contrast bubbles were initially used almost 30 years ago when Gramiak and Shah51 observed that the injection of free gas bubbles produce an enhancement of the ultrasound image. However, it took almost another 20 years of development for the first commercially produced intravenous contrast agent to become available in 1989. The ideal contrast agent should be small and have rheologic properties similar to those of red blood cells. Normal pulmonary capillaries are 5 to 8 μm in diameter; therefore, microbubbles also have to be smaller than this to avoid entrapment in the pulmonary microvasculature.52 However, since the sound-scattering potential of a microbubble is proportional to the sixth power of its radius, the microbubble may not be adequately visualized if it is too small. Thus, the ideal microbubble diameter should be between 4 and 6 μm. Microbubbles also should be stable on intravenous injection, have good in-vivo persistence to allow imaging from multiple imaging planes, and be neutrally buoyant (do not float out of emulsion). They also should be safe, nontoxic, and readily metabolized.
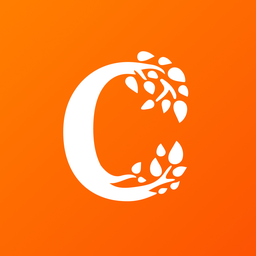
Full access? Get Clinical Tree
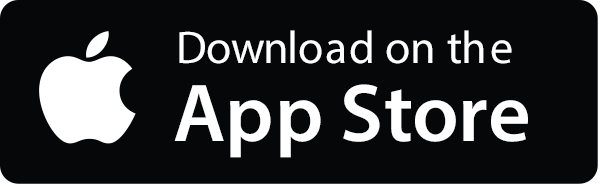
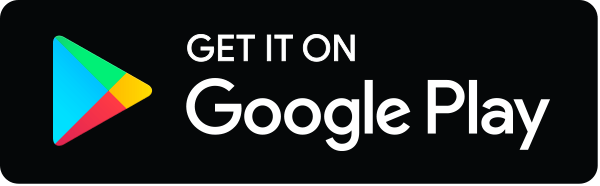