Fig. 40.1
Depiction of electrical activity and the occurrence of irreversible cell death (infarction) as cerebral blood flow is reduced from normal (50 cm3/min/100 g). As shown, the EEG becomes abnormal below 22 cm3/min/100 g and absent when blood flow reaches 15 cm3/min/100 g. Infarction occurs at 17–18 cm3/min/100 g after 3–4 h and progressively shorter periods with blood flow below this level
As shown, cortical electrical activity becomes abnormal below the threshold of adequate blood flow, which is estimated to be about 22 cm3/min/100 g of brain weight and absent below 15 cm3/min/100 g (ischemic threshold) [4]. This ischemia can eventually lead to cellular death given sufficient time, with the time being related to the degree of hypoperfusion. Increasing severity of neural injury is inversely proportional to blood flow, increased severity with decreased flow, and directly proportional to time. Increased severity is seen with increased duration of ischemia. Hence, the fall in electrical activity signals a reduction in blood flow and the need to improve flow to increase the allowable operative time until irreversible injury occurs.
As described below, during TAA repair , improvement in flow may involve increasing blood pressure or reimplantation of blood vessels important for spinal cord perfusion or reducing the cerebrospinal fluid pressure to improve the net perfusion pressure. These maneuvers usually require additional operative time (e.g., reimplanting intercostal arteries), which risks increasing the ischemic time such that IOM can help determine if it is necessary and if it is effective.
Spinal Cord Blood Supply
Because of the role of spinal cord blood flow, it is useful to review the blood supply of the spinal cord. The spinal cord is supplied by the anterior spinal artery (AntSA) , which perfuses the anterior two-thirds to four-fifths of the spinal cord , including the white matter motor tracts and the grey matter containing the anterior horn cells. The AntSA is key to the perfusion of the motor tracts and the MEP. Two posterior spinal arteries (PostSAs) supply the remaining portions of the cord, including the white matter dorsal columns and a small part of the posterior funiculi. The PostSAs are keys to the perfusion of the pathway of the SSEP. The PostSA and to some degree the AntSA run the length of the spinal cord; however, the AntSA is not continuous, especially in the midcervical, upper thoracic, and a narrowed region just cephalad to the lumbar enlargement. An anastomotic vascular ring surrounds the spinal cord and provides some shared blood flow between the anterior and posterior spinal arteries.
The spinal cord blood flow is autoregulated similar to the brain [5]. As such, the normal spinal cord will attempt to maintain spinal cord blood flow over a wide perfusion pressure. In some individuals, autoregulation extends from approximately 50–150 mmHg above the normal cerebrospinal fluid pressure (CSFP) . However, similar to the brain , a wide variation exists, with some individuals having a lower limit of autoregulation that is substantially higher than for most individuals [6]. If the perfusion pressure falls below this lower limit of autoregulation, the spinal cord blood flow becomes directly dependent on perfusion pressure, which inevitably leads to ischemia.
The anterior and posterior spinal arteries are fed by arterial vessels from the aorta along the spinal column. In the cephalad region, they are fed by the vertebral arteries, and as they descend along the spinal cord they receive radicular perforators from the aorta. The paired PostSAs are fed by small radicular arteries at nearly every vertebral body. The AntSA receives blood flow from only two to eight radicular arteries that originate from the aorta [7]. In the cervical region, two or three segmental arteries arise from the cervical or subclavian arteries. The thoracic cord usually has only one to three anterior segmental arteries arising from the aorta, making it particularly susceptible to ischemia; blood flow to the spinal cord is compromised by reductions in pressure due to the long distances between major vessels. The region between T4 and T7 is thought to be the least well-supplied region of the spinal cord, and it is particularly vulnerable to ischemia. This explains the higher risk of paralysis in patients with disease of the thoracic segment of the aorta.
One anterior segmental artery is larger than the others and supplies about 75 % of the blood flow to the AntSA. This artery is referred to as the “arteria radicularis magna ” (ARM or Artery of Adamkiewicz) and supplies the lumbar enlargement of the spinal cord. The location of the ARM is variable, with the artery arising in 75 % of patients from mostly left-sided intercostal arteries at T9–T12.
The vascular anatomy in patients with TAA is highly variable and patients may develop collateral circulation around arteries occluded by mural thrombi or arterial plaques. A meshwork of collateral blood vessels develops with the lumbar arteries (L3–L5) and the pelvic circulation becoming the main blood supply to the spinal cord in almost a quarter of the patients [8, 9]. This meshwork may explain why loss of some radicular arteries may not have the same consequences as in a normal patient. This also explains why the distal perfusion of the aorta is critically important during aorta cross-clamping.
In some patients, the radicular perforators from the PostSAs are critically important to spinal perfusion because of wide spacing between AntSA vessels, creating watershed regions of interrupted and poor perfusion. These critical arteries are most commonly seen between T8 and L4 [7, 8, 10]. In patients who are dependent on these vessels, critical intercostals need to be identified and reimplanted in order to reduce the incidence of operative paraplegia. With the ARM supplying 75 % of blood flow, it is extremely important to reimplant the ARM in patients deemed at risk for loss of other critical vessels [8, 11–13]. Further, when critical radicular arteries are disconnected from the aorta during surgery and not reconnected, retrograde flow may cause a redistribution of blood from the AntSA [14].
In summary, the blood supply to the spinal cord is critically dependent on a meshwork of blood vessels supplied cephalad from the vertebral arteries, at the caudal end from sacral and iliac arteries, and from radicular perforators from intercostal arteries (which include the ARM). No consistent vascular pattern is seen in all patients, and spinal cord dependency on specific vascular regions varies. For example, in some patients, the supply from the cephalad vessels is sufficient such that the entire aorta can be excluded (without reimplantation of intercostals vessels) with no postoperative paraplegia occurring. In others, the key role of the pelvic circulation requires atrial-femoral or femoral venoatrial bypass to provide retrograde distal perfusion. Some patients are critically dependent on specific intercostal vessels. Unfortunately, these vessels cannot be identified using arteriograms. The role of IOM is to assist in identifying the specific ischemic risk factors in each patient and guide the necessary corrective measures to reduce neurological risk.
Open Surgical TAA Repair
Because of the risk from spinal cord, visceral, and limb ischemia, together with renal and respiratory failure, an open surgical repair of a TAA contributes to early mortality and morbidity. The recent American College of Cardiology/American Heart Association Guidelines for the Diagnosis and Management of Patients with Thoracic Aortic Disease gave recommendations for intervention based on the size of the thoracic aorta [15]. Based on these guidelines, open surgery is recommended for patients with TAAs larger than 6 cm (Class I, Level of Evidence: B) and for smaller sized aneurysms in patients with connective tissue disorders (Class I, Level of Evidence: C) [16]. Surgical repair is also recommended when there is end-organ ischemia or significant celiac, superior mesenteric, or renal artery atherosclerosis (Class I, Level of Evidence: B) [3]. Acute dissection involving the ascending aorta needs swift open surgical repair, although in some cases, hybrid approaches or endovascular interventions can be used [3, 17]. Finally, symptomatic aneurysms should be resected regardless of size [18].
Monitoring Strategy During Open TAA Surgery
To understand the role of IOM , it is useful to consider the surgical procedure in stages and consider the strategy for use of IOM so as to reduce the risk of paralysis [19]. Initially, proximal cross-clamping of the aorta is done. At this point, all spinal cord perfusion comes from the vertebral arteries. This cross-clamp also causes a critical elevation of blood pressure proximal to the clamp with accompanying elevations in cerebrospinal fluid (CSF) pressure in the brain and spinal cord. This increased CSF pressure can further reduce spinal cord perfusion pressure (SCPP) [SCPP = spinal arterial pressure − spinal CSF pressure] compounding the reduction of spinal cord blood flow [2]. In order to minimize hypoperfusion, many surgeons try to limit the aortic cross-clamp to no more than 30–40 min.
Since perfusion is often not adequate, a surgeon may place a shunt or bypass pump between the proximal and distal aorta to extend the safe operative time. This bypass reduces the proximal hypertension and the associated increased CSFP . It provides better distal spinal cord perfusion for patients who are dependent on the caudal vessels [8]. Although a bypass perfusion pressure of 60–70 mmHg is generally accepted as adequate, the pressure necessary to effectively perfuse critical vessels is not known in any given patient without functional testing provided by IOM. Adequate pressure where IOM studies maintain pre-cross-clamp amplitude and latency is as high as 90–110 mmHg [20, 21]. Use of these distal perfusion techniques has been reported to reduce the risk of paralysis to about 10 % from the risk of 30–50 % with cross-clamping alone [14].
In some patients, distal perfusion alone is adequate to provide spinal cord perfusion. In other patients, perfusion from the critical radicular arteries is also required to prevent paraplegia. In more than half of the patients with aneurysms, a rich collateral network surrounding the spinal cord prevents ischemia, even when otherwise critical intercostal arteries are sacrificed [22]. But a group of patients with inadequate collateral networks may exist, in which reimplantation of intercostal arteries may be crucial for maintaining adequate spinal cord blood flow. These patients can be identified using IOM testing during segmental cross-clamping of the aorta.
Several methods are available to provide reperfusion of critical arteries once they are identified. One approach is to preserve the back wall of the aneurysm where the intercostal arteries arise and use it in the aortic reconstruction thus restoring critical perfusion [2]. Since this technique may not be feasible, other techniques have been developed to identify and implant the arteries in the repaired aorta segment. Because of its importance, some surgeons attempt to identify the ARM preoperatively to ensure that it is reimplanted into the aortic graft. Simply reimplanting the ARM further reduces the risk of paralysis from 10 % with distal perfusion to 5–6 % [14, 23]. If it cannot be identified, some surgeons reimplant all intercostals in the T8–T12 range [2].
Intraoperative monitoring can also be useful to help assess if other techniques employed to reduce the risk of spinal cord ischemia are useful. Of particular note is the use of CSF drainage to maintain a low CSFP and improve the net perfusion pressure (CSFPP). Intraoperative monitoring can assist in identifying the critical CSFP that improves perfusion [24, 25].
In short, the goal of the surgical technique is to optimize the perfusion and reduce the overall ischemic time of the spinal cord. Hence, the surgeon must work expeditiously during the cross-clamp time. The value of IOM is to promptly identify spinal cord regions that are ischemic while the insult is reversible and then guide the interventions to correct the ischemia and reduce the risk. IOM has been applied successfully to these surgeries and has reduced the risk of intraoperative paralysis.
Attempts to increase the allowable ischemic time in TAA repair by using hypothermia have not been universally applied because it can lead to adverse systemic effects of prolonged cardiopulmonary bypass and profound hypothermia (e.g., coagulopathy, endothelial dysfunction, systemic inflammation) when applied systemically [26]. However, since deep hypothermia (14.1–20.0 °C) can reliably protect the spinal cord from ischemic damage, techniques using regional hypothermia are being explored. The results in early reports of TAA repair under regional spinal cord hypothermia using a custom-designed epidural catheter were excellent [26, 27]. Unfortunately, an ideal agent for pharmacologic neuroprotection has yet to be found [27].
Application of Intraoperative Monitoring to TAA Surgery
Since SSEP monitoring has been available since the 1980s, the most extensive clinical experience has been with SSEP monitoring. During aortic surgery, use of this technique can identify ischemia of the neural tract including peripheral nerve, white matter tracts of the dorsal columns, and ischemia in the brainstem or cerebral cortex.
A slow onset of cortical SSEP change (>15 min) usually indicates peripheral nerve ischemia. Isolated events, such as the femoral artery bypass cannula occluding flow to the leg, is one of the most frequent causes of peripheral nerve ischemia and unilateral loss of SSEP. If a bilateral change occurs within 15 min, it has been assumed to be of spinal cord origin, either from inadequate distal aorta perfusion or loss of critical intercostals. Thus, clinicians have used the SSEP to determine if bypass was necessary, if the pressure of bypass was adequate, and if it was safe to exclude a specific radicular artery.
One of the earliest human studies using the SSEP was done by Cunningham and Laschinger et al. [28]. They observed four patterns of change in the SSEP depending on the mechanism of ischemia. A type I pattern was where the SSEP was altered in 3–4 min following proximal cross-clamping of the aorta with complete loss in 8–9 min. This pattern was thought to be indicative of inadequate perfusion of the spinal cord from the distal arterial supply. This emphasized the value of bypass perfusion [29]. A type II pattern was where the SSEP did not change after aortic cross-clamping, suggesting adequate spinal perfusion was present from cephalad vessels.
A type III pattern was where the patient was critically dependent on a radicular artery and changes in the SSEP were noted when it was occluded. This points out the use of the SSEP to locate the critical intercostals during aortic cross-clamping. The SSEP was shown to be restored when the critical intercostal was reimplanted or unclamped. Other studies report similar changes; rapid reperfusion of critical intercostal vessels restores the cortical SSEP and appears to reduce the risk of paraplegia [28, 30, 31]. A type IV pattern, where a gradual “fade out” reduction in amplitude but not latency of the SSEP over 30–50 min, is considered characteristic of lower extremity hypoperfusion [32]. This may signal the need to reorient the bypass cannula.
Use of the SSEP has not been completely reliable for always being able to predict paralysis since it does not monitor the motor tracts and instead monitors proprioception and vibration pathways in the posterior spinal cord [20]. In some cases, an SSEP loss has been correlated with motor injury. This is seen most often when an SSEP loss occurred rapidly after cross-clamping (within 3–5 min) or when the duration of an SSEP loss was prolonged (40–60 min) [29, 33–35].
With the advent of reliable motor-evoked potential monitoring, clinical inquiry turned toward the use of transcranial motor-evoked potential (MEP) monitoring. This form of monitoring differs from SSEP monitoring in that the responses can detect ischemia in the anterolateral white matter tracts, the spinal grey matter, and the peripheral nerve and muscles. The use of MEPs is of particular interest since aortic occlusion in dogs demonstrated that the predominant injury from spinal cord ischemia is grey matter necrosis [36, 37].
Although false negatives with MEP monitoring have occurred (i.e., immediate postoperative paralysis with preserved intraoperative MEP), most studies show an excellent correlation of outcome with MEP findings [8, 13, 20, 21, 25, 32, 38]. All studies, both clinical and experimental, have reported a rapid change in MEP, within 2–4 min, to ischemic conditions where the ischemia includes the spinal grey matter serving the muscles monitored. This rapid response provides more useful feedback in the intraoperative environment where time is critical in determining outcome. These clinical studies suggest that MEP monitoring is more sensitive to ischemia than SSEP monitoring and provides significant additional reductions in paraplegia.
Comparison of SSEP and MEP in Aorta Surgery
Clinical studies have found that when comparing SSEP and MEP findings, there is a relatively long delay (7–30 min) between the onset of ischemia and the disappearance of SSEP, whereas the MEP generally changes comparatively quickly within 2–5 min [21, 32, 39–41]. The most important finding was that SSEP changes were not always associated with MEP changes.
Clearly, the effectiveness of the monitoring and some of the differences between the SSEP, MEP, and epidural recorded responses relate to the specific neural tracts being monitored and their susceptibility to ischemia. Based on the available data, the time to electrical failure of various neural tissues is shown in Table 40.1. The cerebral cortex is the most sensitive to ischemia, with a loss of electroencephalographic (EEG) activity within 20 s after inadequate perfusion. In the spinal cord, the grey matter is most sensitive to ischemia, with a loss of synaptic activity within 1–2 min. Conduction in sensory and motor white matter tracts (axons) demonstrates alterations in activity within 3–6 min (SSEP tract) or 11 min (motor tract) and a loss of conduction at between 7 and 18 min (SSEP) or 11 and 17 min (MEP). Hence, the white matter tracts of the sensory and motor systems are about equal to each other in terms of sensitivity to ischemia [20, 32, 42, 43]. Isolated peripheral nerve ischemia results in the loss of SSEP and MEP conduction after 20–30 min.
Table 40.1
Time to electrical failure in selected spinal neural tissues
Tissue | Time to electrical failure |
---|---|
Cerebral cortex | 20 s |
Spinal grey matter | 1–2 min |
White matter (sensory) | 7–18 min |
White matter (motor) | 11–17 min |
Peripheral nerve | 20–45 min |
The second issue comparing SSEP to MEP pertains to the prediction of outcome. When focusing on motor outcome, the MEP has the greater predictive power because it actually measures function in the cortical-spinal tract (CST). However, since only 5 % of the CST fibers are involved in the response, the correlation is not exact [44]. Further, a variety of other descending tracts are needed for coordinated motor function, so losses in associated tracts may hamper motor function despite maintenance of adequate motor cell function in the CST [44]. Use of SSEPs also has the drawback that ischemia in the anterior spinal region that supplies the motor tracts may not be reflected in the posteriorly located SSEP tracts. Thus, the differences in arterial blood supply, AntSA versus PostSAs, produce a differential vulnerability to ischemia. Hence, the loss of the SSEP and MEP acts as a surrogate for the entire functional aspect of the spinal cord.
The progression of spinal cord ischemia after TAA interventions can frequently be identified by IOM and arrested before irreversible infarction results [27]. This spinal cord rescue depends on the early detection and immediate multimodal intervention to maximize spinal cord oxygen supply. The routine application of IOM in TAA repair remains controversial, although many operative teams have successfully integrated it into their practice [15]. Some studies show a significant role of MEPs during TAA repair and the subsequent surgical strategies necessary to maintain or regain spinal cord function, leading to significantly reduced paraplegia rates [45]. The recent American College of Cardiology/American Heart Association Guidelines for the Diagnosis and Management of Patients with Thoracic Aortic Disease indicate that motor- or somatosensory-evoked potential monitoring can be useful when the data will help to guide therapy (CLASS IIa, Level of Evidence: B) [16]. Protocols to guide therapy include those mentioned above (e.g., CSF drainage, increase in mean arterial and distal aortic pressure, reattachment of intercostal arteries) [45].
Endovascular Stent Placement
Although open surgical repair is the superior technique, thoracic endovascular aneurysm repair (TEVAR) avoids subjecting patients whose comorbid conditions predispose them to significant morbidity or mortality to the open procedure (especially a thoracotomy) [46]. Endovascular grafting may be of particular value in patients with significant comorbid conditions (older age, substantial cardiac, pulmonary, and renal dysfunction) who would be considered poor or unacceptable candidates for open surgery [16]. Recommendations from the American College of Cardiology/American Heart Association Guidelines for the Diagnosis and Management of Patients with Thoracic Aortic Disease are that in patients with degenerative or traumatic aneurysms of the descending thoracic aorta exceeding 5.5 cm, saccular aneurysms, or postoperative pseudoaneurysms, endovascular stent grafting should be strongly considered when feasible (CLASS I, Level of Evidence: B) [3, 16].
The potential advantages of endovascular grafting include the absence of a thoracotomy incision and the need for partial or total extracorporeal circulatory support and clamping of the aorta. This procedure also has the advantage of a shorter ischemic time for distal tissues. In addition, several factors increase the blood flow reserve of the spinal cord during the perioperative period. These include (1) an increased cardiac output, (2) increased blood pressure, (3) avoidance of hypoxemia and anemia, (4) preservation of the critical intercostal arteries, and (5) reduced CSF pressure [21, 47].
The clinical advantages of endovascular stent placement have been stated to include shorter intensive care unit and hospital stay, lower 30-day mortality, fewer pulmonary complications, reduced pain, and reduced transfusion requirements [46, 48]. For example, 50 % of patients have chronic obstructive pulmonary disease, and the reduced pulmonary complications associated with stent placement improve the likelihood of hospital discharge [46].
However, endovascular treatment of TAAs is significantly more complex due to the need to incorporate visceral arteries in the repair at the same time that the aneurysm is excluded. One major problem with the endovascular techniques is the limited ability to provide vascular supply to blood vessels originating from the aorta in the region of the stent. This has led to stents with branches or fenestrations to provide a means to supply vessels such as the renal or mesenteric arteries [46]. The stents have not, however, been well developed for providing flow to the ARM or critical radicular arteries, such that spinal cord ischemia could occur if these vessels are compromised and are essential. These stents may require 4–6 weeks of manufacturing time, making them less useful in an emergency or for an urgent repair [15].
Several factors may limit the ability to use TEVAR in an individual patient. These include the absence of suitable “landing zones” above and below the aneurysm (usually 2–3 cm of normal diameter aorta without circumferential thrombus for the stent to attach), an aortic width at the landing zones that exceeds the recommended width for the largest available endovascular grafts (generally 10–15 % larger than the width of the aorta), a lack of vascular access sites, and severe aortic atherosclerosis [3, 16].
The disadvantages of TEVAR include late complications, which are uncommon with the open technique (graft migration, stent fracture, endovascular leaks, and stent failure necessitating surgical correction) [48]. In addition, TEVAR is associated with increased risk of atheroembolic stroke and retrograde type A dissection (especially in patients with fragile aortic wall) [49].
Although the complications of the open portion of the procedure are reduced, there is evidence that endovascular treatment of TAAs, similarly to open repair, carries the same risks of paraplegia, renal failure, stroke, and death [15]. High-risk factors of spinal cord injury during endovascular aortic repair are (1) coverage of the left subclavian artery, (2) extensive coverage of long segments of the thoracic aorta, (3) prior downstream aortic repair, (4) compromising important intercostal (T8–L1), vertebral, pelvic, and hypogastric collaterals, and (5) an aorta prone to give off atheromatous emboli [47].
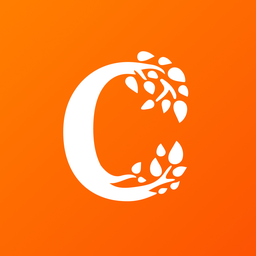
Full access? Get Clinical Tree
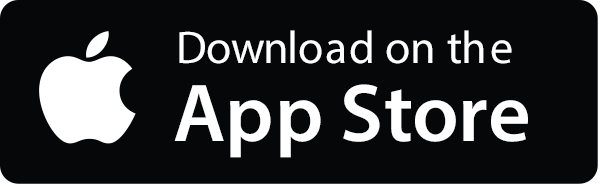
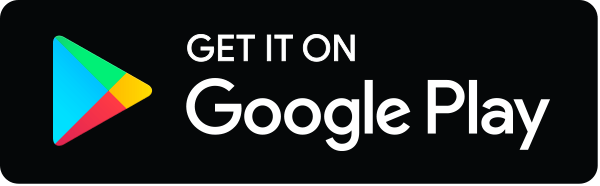