Chapter 39
Electrolyte Disorders 
Electrolyte disturbances are common among patients in intensive care units (ICUs). Anticipation and prompt recognition of these disorders are crucial skills for the physician providing care for critically ill patients. Appropriate treatment depends on an understanding of the underlying pathophysiologic processes, as well as an appreciation of the often complex and multisystem disorders affecting these patients. This chapter addresses electrolyte disorders of potassium, calcium, magnesium, and phosphorus. Separate chapters address acid-base disorders (Chapters 82 and 83) and disorders of water homeostasis and the serum sodium concentration (Chapter 84).
Potassium Disorders
Hyperkalemia
Box 39.1 lists common acute causes of hyperkalemia relevant to the ICU setting. Before pursuing a diagnosis of hyperkalemia, it is important to consider pseudohyperkalemia, when an elevation in measured serum potassium concentration is due to potassium movement out of cells into the serum during or after the blood specimen has been obtained. In pseudohyperkalemia, the true potassium concentration in blood and plasma is normal, but when measured in the serum by the clinical laboratory, an elevated value is reported. Major causes of pseudohyperkalemia include hemolysis resulting from mechanical trauma during venipuncture, severe thrombocytosis (> 400,000/mm3) or leukocytosis (> 100,000/mm3), repeated fist clenching during blood drawing (resulting from the release of potassium by exercising muscle), and, less commonly, hereditary spherocytosis and familial pseudohyperkalemia. Diagnosis of pseudohyperkalemia caused by the release of intracellular potassium from platelets or white blood cells within the specimen container can be made by obtaining a plasma (unclotted) potassium concentration, which is normally < 0.5 mmol/L lower than the serum potassium concentration.
Multiple factors are necessary to overcome the several autoregulatory mechanisms protective of the extracellular potassium concentration, in order to produce acute or chronic hyperkalemia. Homeostatic mechanisms maintain balance between intracellular and extracellular potassium (internal balance) and control renal (and to a much lesser extent gastrointestinal [GI] tract) potassium excretion (external balance) to prevent development of significant hyperkalemia even despite large increases in potassium intake. Consequently, clinically significant sustained hyperkalemia generally requires both disturbed internal balance and impaired renal excretion. Impaired adrenal function or impaired responsiveness to the effect of adrenal hormones, particularly aldosterone, is also often present, as a consequence of intrinsic adrenal gland disease or medications that inhibit aldosterone synthesis or interfere with the renin-angiotensin-aldosterone system (see Box 39.1).
Clinical Manifestations
Because of its critical role in establishing transmembrane potential, elevations in serum potassium concentration affect cardiac membrane potential and neuromuscular transmission with related clinical effects predominantly involving the myocardium and skeletal muscles. The earliest manifestation, usually seen when potassium concentration is above 5.5 to 6 mmol/L, is peaking of the T waves on the electrocardiogram (ECG) with shortening of the QT interval (Figure 39.E1A) . More severe hyperkalemia (i.e., > 6 mEq/L) is first associated with prolongation of the PR interval and QRS complex duration. As the potassium concentration rises further, the P wave may be lost entirely, the QRS complex widens further and merges with the T wave producing a “sine wave” pattern; ventricular fibrillation or ventricular standstill with cardiac arrest may occur (Figure 39.E1B
). However, serum potassium concentration poorly correlates with observed ECG changes in hyperkalemia, and the effect of hyperkalemia on myocardium is influenced by acidosis, hyponatremia, and hypocalcemia, all of which increase the neuromuscular effects of hyperkalemia. Careful monitoring and prompt treatment of patients with even minor ECG findings are essential.
Treatment
Prompt reversal of hyperkalemia and prevention of its cardiac effects are necessary to mitigate the life-threatening nature of hyperkalemia-induced cardiac arrhythmias. In all hyperkalemic patients, excessive potassium intake in the diet, medications, and enteral or parenteral nutrition should be eliminated. If possible, discontinue medications that impair urinary potassium excretion (see Box 39.1). In the setting of the previous ECG or other neuromuscular manifestations, treatment should proceed in three phases.
2 Shift of Potassium into Cells
By buffering extracellular H+ and raising blood pH, sodium bicarbonate given as 50 mL of 7.5% solution intravenously over several minutes can lead to an exchange of intracellular hydrogen for extracellular potassium. Intravenous sodium bicarbonate is not as effective as insulin or beta-agonists in lowering the serum potassium concentration, especially in the absence of severe acidosis, and risks volume overload and hypernatremia. In addition, the hypertonic nature of the solution will cause water movement from the intracellular to extracellular space, carrying via convection the intracellular potassium concentration of 120 meq/L, thus potentially increasing serum potassium concentration. As such, the use of IV sodium bicarbonate should be limited to patients with hyperkalemia and concomitant severe acidemia.
Hypokalemia
Hypokalemia can develop from an internal shift of potassium from the extracellular to intracellular spaces without a deficiency of total body potassium. More commonly, hypokalemia results from total body potassium deficits that can be in the range of several hundreds of mmol because of excessive losses via the GI tract, kidneys, or both, often exacerbated by inadequate oral intake. Box 39.2 lists important causes of hypokalemia (serum potassium concentration < 3.5 mmol/L) that are commonly seen in ICU patients. Hypokalemic patients with primarily extrarenal potassium losses and intact renal resorptive capacity should have daily urinary potassium excretion < 20 mmol and urinary potassium concentration < 10 mmol/L. Levels exceeding these values suggest at least some element of urinary potassium wasting.
Clinical Manifestations
Even though hyperkalemia has primarily neuromuscular and cardiac effects, hypokalemia has more varied effects impacting multiple organ systems, although patients with mild hypokalemia or hypokalemia that develops slowly may be asymptomatic.
Gastrointestinal symptoms include constipation that can progress to paralytic ileus, depending on the severity of the hypokalemia. Musculoskeletal effects range from mild generalized weakness, cramps, paresthesias, and myalgias with mild hypokalemia to loss of deep tendon reflexes, rhabdomyolysis, and skeletal and respiratory muscle paralysis at serum potassium concentration < 2 to 2.5 mmol/L. ECG changes include ST segment depression, reduced T-wave amplitude, and development of prominent U waves. Cardiac arrhythmias associated with hypokalemia include sinus bradycardia, paroxysmal tachycardia, atrioventricular blocks, premature atrial and ventricular beats, and ventricular tachycardia and fibrillation. Use of digitalis glycosides, concomitant cardiac ischemia, and hypomagnesemia potentiate the likelihood of hypokalemia-associated cardiac arrhythmias. Hypokalemia also impairs urinary concentrating ability with symptoms of nocturia and polyuria because of nephrogenic diabetes insipidus and increases renal ammoniagenesis, which can precipitate or worsen hepatic encephalopathy. It augments renal hydrogen ion (H+) excretion, promoting development of metabolic alkalosis (Chapter 83). If long-standing and severe, hypokalemia can cause renal interstitial fibrosis, tubular atrophy, and renal cyst formation associated with reduced glomerular filtration rate and chronic kidney disease (CKD).
Treatment
Take care during repletion of critically ill patients with long-standing hypokalemia, as they may not be able to rapidly shift administered potassium loads intracellularly. Overly aggressive intravenous repletion can result in dangerous hyperkalemia. Therefore, oral or enteral repletion with 40 mmol of elemental potassium as potassium chloride capsules, tablets, or elixir (potassium citrate or potassium bicarbonate may be given in patients with metabolic acidosis) is safest for mild and moderate hypokalemia. Monitor the serum potassium concentration every 4 to 6 hours and administer repeat doses as needed until the potassium concentration normalizes and remains within the normal range. The serum potassium concentration can acutely rise by as much as 1.5 mmol/L after an oral dose of 60 meq but will typically subsequently decline because of cellular potassium uptake until the total body potassium deficit has been corrected.
Calcium Disorders
Changes in the serum albumin concentration are associated with parallel changes in total serum calcium concentration. A commonly used approximation is that for each 1 g/dL decrease in albumin concentration below 4.5, the total serum calcium would be expected to fall by 0.8 mg/dL. This relationship allows clinicians to estimate the “corrected” total serum calcium accounting for the presence of hypoalbuminemia. Systemic pH and other factors (serum phosphate concentration, PTH level) can also influence the ionized calcium concentration. Acidemia decreases calcium binding to albumin and increases the fraction of total calcium that is in the ionized form. Conversely, alkalemia decreases the ionized calcium concentration by increasing the binding of calcium to albumin. For instance, acute respiratory alkalosis causes the ionized calcium concentration to decline by approximately 0.16 mg/dL (0.04 mmol/L) for each 0.1 unit increase in pH. Direct measurement of ionized calcium is possible in most clinical laboratories and should be employed in any patient with signs or symptoms concerning for hyper- or hypocalcemia with a normal total serum calcium concentration and in patients in whom serum total calcium measurements may be unreliable because of the presence of factors mentioned previously.
Hypercalcemia
Box 39.3 lists some of the more common causes of hypercalcemia relevant to the ICU setting. Asymptomatic or mildly symptomatic hypercalcemia, particularly if relatively chronic, is more likely to be due to hyperparathyroidism, whereas severe and acute hypercalcemia is more often a complication of underlying malignancy. Appropriate initial workup includes a careful review of the diet and medication list (prescription, over-the counter, vitamin and calcium supplements) as well as measurement of the intact PTH level. Further diagnosis should proceed according to the clinical presentation and may include measurement of serum phosphate, 25-(OH) vitamin D, 1,25-(OH)2 vitamin D level, PTH-related protein, serum and urine protein electrophoreses, and evaluation for malignancy.
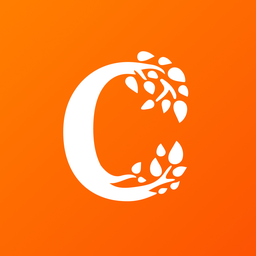
Full access? Get Clinical Tree
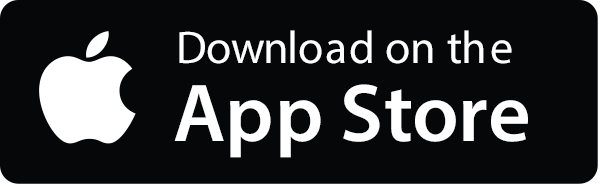
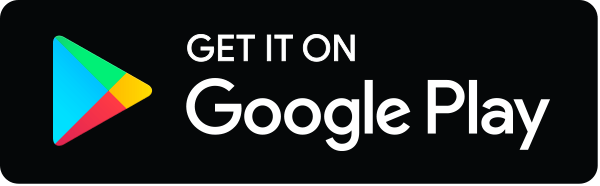