Key Points
- 1.
The electrocardiogram reflects differences in transmembrane voltages in myocardial cells that occur during depolarization and repolarization within each cycle.
- 2.
Processing of the electrocardiogram occurs in a series of steps.
- 3.
Where and how electrocardiographic (ECG) electrodes are placed on the body are critical determinants of the morphology of the ECG signal.
- 4.
ECG signals must be amplified and filtered before display.
- 5.
How accurately the clinician places ECG leads on the patient’s torso is probably the single most important factor influencing clinical utility of the electrocardiogram.
- 6.
The ST segment is the most important portion of the QRS complex for evaluating ischemia.
- 7.
Use of inferior leads (II, III, aVF) allows superior discrimination of P-wave morphology and facilitates visual diagnosis of arrhythmias and conduction disorders.
- 8.
Electrolyte abnormalities typically cause changes in repolarization (ST-T-U waves).
Despite the introduction of more sophisticated cardiovascular monitors such as the pulmonary artery catheter and echocardiography, the electrocardiogram (coupled with blood pressure measurement) serves as the foundation for guiding cardiovascular therapeutic interventions in the majority of anesthetic cases. It is indispensable for diagnosing arrhythmias, acute coronary syndromes, and electrolyte abnormalities (particularly of serum potassium and calcium) and in the detection of some forms of genetically mediated electrical or structural cardiac abnormalities (eg, Brugada syndrome).
One of the most important changes in electrocardiography is the widespread use of computerized systems for recording electrocardiograms. Bedside units are capable of recording diagnostic-quality 12-lead electrocardiograms that can be transmitted over a hospital network for storage and retrieval. Most of the electrocardiograms in the United States are recorded by digital, automated devices, equipped with software that can measure electrocardiographic (ECG) intervals and amplitudes and can provide virtually instantaneous interpretation.
The 12-Lead System
Where and how ECG electrodes are placed on the body are critical determinants of the morphology of the ECG signal. Lead systems have been developed based on theoretical considerations and references to anatomic landmarks that facilitate consistency among individual patients (eg, standard 12-lead system). Einthoven established electrocardiography using three extremities as references: the left arm (LA), right arm (RA), and left leg (LL). He recorded the difference in potential between the LA and RA (lead I), between the RA and LL (lead II), and between the LA and LL (lead III) ( Fig. 9.1 ). Because the signals recorded were differences between two electrodes, these leads were called bipolar. The right leg (RL) served only as a reference electrode. Because the Kirchhoff loop equation states that the sum of the three voltage differential pairs must equal zero, the sum of leads I and III must equal lead II.

The positive or negative polarity of each of the limbs was chosen by Einthoven to result in positive deflections of most of the waveforms and has no innate physiologic significance. He postulated that the three limbs defined an imaginary equilateral triangle with the heart at its center. Wilson refined and introduced the precordial leads into clinical practice. To implement these leads, he postulated a mechanism whereby the absolute level of electrical potential could be measured at the site of the exploring precordial electrode (the positive electrode). A negative pole with zero potential was formed by joining the three limb electrodes in a resistive network in which equally weighted signals cancel each other out. He called this the central terminal, and in a fashion similar to Einthoven’s vector concepts, he postulated that it was located at the electrical center of the heart, representing the mean electrical potential of the body throughout the cardiac cycle. He described three additional limb leads (aVL, aVR, and aVF) . These leads measured new vectors of activation, and in this way the hexaxial reference system for determination of the electrical axis was established. He subsequently introduced the six unipolar precordial V leads in 1935.
Six electrodes are placed on the chest in the following locations: V 1 , fourth intercostal space at the right sternal border; V 2 , fourth intercostal space at the left sternal border; V 3 , midway between V 2 and V 4 ; V 4 , fifth intercostal space in the midclavicular line; V 5 , in the horizontal plane of V 4 at the anterior axillary line, or, if the anterior axillary line is ambiguous, midway between V 4 and V 6 ; and V 6 , in the horizontal plane of V 4 at the midaxillary line (see Fig. 9.1 ).
The 12-Lead System
Where and how ECG electrodes are placed on the body are critical determinants of the morphology of the ECG signal. Lead systems have been developed based on theoretical considerations and references to anatomic landmarks that facilitate consistency among individual patients (eg, standard 12-lead system). Einthoven established electrocardiography using three extremities as references: the left arm (LA), right arm (RA), and left leg (LL). He recorded the difference in potential between the LA and RA (lead I), between the RA and LL (lead II), and between the LA and LL (lead III) ( Fig. 9.1 ). Because the signals recorded were differences between two electrodes, these leads were called bipolar. The right leg (RL) served only as a reference electrode. Because the Kirchhoff loop equation states that the sum of the three voltage differential pairs must equal zero, the sum of leads I and III must equal lead II.
The positive or negative polarity of each of the limbs was chosen by Einthoven to result in positive deflections of most of the waveforms and has no innate physiologic significance. He postulated that the three limbs defined an imaginary equilateral triangle with the heart at its center. Wilson refined and introduced the precordial leads into clinical practice. To implement these leads, he postulated a mechanism whereby the absolute level of electrical potential could be measured at the site of the exploring precordial electrode (the positive electrode). A negative pole with zero potential was formed by joining the three limb electrodes in a resistive network in which equally weighted signals cancel each other out. He called this the central terminal, and in a fashion similar to Einthoven’s vector concepts, he postulated that it was located at the electrical center of the heart, representing the mean electrical potential of the body throughout the cardiac cycle. He described three additional limb leads (aVL, aVR, and aVF) . These leads measured new vectors of activation, and in this way the hexaxial reference system for determination of the electrical axis was established. He subsequently introduced the six unipolar precordial V leads in 1935.
Six electrodes are placed on the chest in the following locations: V 1 , fourth intercostal space at the right sternal border; V 2 , fourth intercostal space at the left sternal border; V 3 , midway between V 2 and V 4 ; V 4 , fifth intercostal space in the midclavicular line; V 5 , in the horizontal plane of V 4 at the anterior axillary line, or, if the anterior axillary line is ambiguous, midway between V 4 and V 6 ; and V 6 , in the horizontal plane of V 4 at the midaxillary line (see Fig. 9.1 ).
Electrocardiographic Artifact
Electrical Power–Line Interference
Electrical power–line interference (60 Hz) is a common environmental problem. Power lines and other electrical devices radiate energy that can enter the monitor by poor electrode contact or cracked or poorly shielded lead cables. Interference can also be induced electromagnetically as these signals radiate through the loop formed by the body, lead cables, and monitor. A line frequency “notch” filter is often used to remove 60-Hz noise.
Electrocautery
Electrocautery units generate radiofrequency currents at very high frequencies (800–2000 kHz) and high voltages (1 kV, which is 100 times greater than the ECG signal). Older units used a modulation frequency of 60 Hz, which spread substantial electrical noise into the QRS frequency range of the ECG signal. Newer units use a modulation frequency of 20 kHz, thus minimizing this problem; however, reports still exist of electrocautery as a cause of artifactual ST-segment changes in intraoperative electrocardiograms. To minimize electrocautery artifact, the RL reference electrode should be placed as close as possible to the return plate, and the ECG monitor should be plugged into a different power outlet from the electrosurgical unit.
Clinical Sources of Artifact
Clinical devices in physical contact with the patient, particularly through plastic tubing, may at times cause clinically significant ECG artifact. Although the exact mechanism is uncertain, two leading explanations are either a piezoelectric effect secondary to mechanical deformation of the plastic or buildup of static electricity between two dissimilar materials, especially those materials in motion (as in the case of cardiopulmonary bypass [CPB] tubing and the roller pump head). In this situation, the electricity generated in the pump flows into the patient through the tubing and is picked up by the electrodes. This artifact is not related to the electricity used to power the CPB pump because it has been reproduced by manually turning the pump heads.
ECG interference during CPB has been recognized for many years. It is manifested by marked irregularity of the baseline, similar to ventricular fibrillation, with a frequency of 1 to 4 Hz and a peak amplitude up to 5 mV. Uncorrected, it may make effective diagnosis of arrhythmias and conduction disturbances very difficult ( Fig. 9.2 ), especially during the critical period of weaning from CPB, and it may also make accurate determination of asystolic arrest from cardioplegia difficult. Accumulation of static electricity is assumed to be the major etiologic factor. Khambatta and colleagues recommended maintaining ambient temperature higher than 20°C.
Electrocardiographic Changes With Myocardial Ischemia
Detection of Myocardial Ischemia
The ST segment is the most important portion of the QRS complex for evaluating ischemia. It may come as a surprise that no gold standard criteria exist for the ECG diagnosis of myocardial ischemia. Many anesthesiologists, when evaluating an electrocardiogram for signs of ischemia, look for signs of repolarization or ST-segment abnormalities. Many other signs of myocardial ischemia may also be seen in the electrocardiogram, including T-wave inversion, QRS and T-wave axis alterations, R- or U-wave changes, or the development of previously undocumented arrhythmias or ventricular ectopy. None of these, however, is as specific for ischemia as ST-segment depression or elevation. Depending on the location of the infarction, and the observed leads, the ST-segment changes have a specificity of 84% to 100% and a sensitivity of 12% to 66% for myocardial ischemia.
The origin of the ST segment, at the J point, is easy to locate. However, J-point termination, which is generally accepted as the beginning of any change of slope of the T wave, is more difficult to determine. Physiologically normal persons may have no discernible ST segment because the T wave starts with a steady slope from the J point, especially at rapid heart rates. The TP segment has been used as the isoelectric baseline from which changes in the ST segment are evaluated, but with tachycardia, this segment is eliminated, and, during exercise testing, the PR segment is used. The PR segment is used in all ST-segment analyzers.
Repolarization of the ventricle proceeds from the epicardium to the endocardium, opposite to the vector of depolarization. The ST segment reflects the midportion, or phase 2, of repolarization during little change in electrical potential. It is usually isoelectric. Ischemia causes a loss of intracellular potassium, resulting in a current of injury. With subendocardial injury, the ST segment is depressed in the surface leads. With epicardial or transmural injury, the ST segment is elevated ( Figs. 9.3 and 9.4 ). Although myocardial ischemia may manifest in PR-segment, QRS complex, ST-segment, or T-wave changes, the earliest ECG signs of ischemia are typically T-wave and ST-segment changes. With myocardial ischemia, repolarization is affected, resulting in downsloping or horizontal ST-segment depression. Various local effects and differences in vectors during repolarization result in different ST-segment morphologic features that are recorded by the different leads. It is generally accepted that ST-segment changes in multiple leads are associated with more severe degrees of coronary artery disease (CAD).
The criteria for myocardial infarction (MI) are divided into two categories: ST-segment elevation MI (STEMI), and ST-segment depression/T-wave change MI (NSTEMI). The J point is located at the juncture of the QRS complex and the ST segment, and it is used to measure the magnitude of the ST-segment deflection as compared with the baseline of the electrocardiogram. A new J-point elevation of 0.1 mV or greater is required in all leads except V 2 and V 3 to meet the criteria for STEMI. J-point elevations of up to 0.25 mV may be seen in leads V 2 and V 3 in healthy men younger than 40 years of age; however, this finding decreases with age and is less prominent in women. The J-point elevations must be seen in two or more contiguous leads for the satisfaction of ST-segment elevation criteria. New horizontal or downsloping ST-segment depressions of 0.05 mV or greater or T-wave inversion of 0.1 mV or greater in two contiguous leads with an R-wave–to–S-wave ratio greater than 1 satisfy the criteria for NSTEMI. However, ST-segment elevations are more specific than ST-segment depressions and/or T-wave inversions for localizing the site of ischemia. ST-segment elevation generally suggests greater degrees of myocardial damage than ST-segment depression or T-wave changes. Previously inverted T waves may pseudonormalize during episodes of acute myocardial ischemia ( Appendix 9.1 ).
Nonspecific ST-segment depression can be related to drug use, particularly digoxin. Interpretation of ST-segment changes in patients with left ventricular hypertrophy is particularly controversial given the tall R-wave baseline, J-point depression, and steep slope of the ST segment.
ST-segment elevation is rarely reported in the setting of noncardiac surgical procedures. However, it is commonly observed during weaning from CPB in cardiac operations and during CABG procedures (on and off pump) with interruption of coronary flow in a native or graft vessel. ST-segment elevation in a lead with a Q wave should not be analyzed for acute ischemia, although it may indicate the presence of a ventricular aneurysm.
Anatomic Localization of Ischemia With the Electrocardiogram
As noted earlier, ST-segment depression is a common manifestation of subendocardial ischemia. From a practical clinical standpoint, it has a single major strength and limitation. Its strength is that it is almost always present in one or more of the anterolateral precordial leads (V 4 –V 6 ). However, it fails to “localize” the offending coronary lesion and has little relation to underlying segmental asynergy.
In contrast, ST-segment elevation correlates well with segmental asynergy and localizes the offending lesion relatively well. Reciprocal ST-segment depression often is present in one or more of the other 12 leads. In patients with angiographically documented single-vessel disease, ST-segment elevation (as well as Q waves or inverted T waves) in leads I, aVL, or V 1 through V 4 is closely correlated with disease of the left anterior descending coronary artery, whereas similar findings in leads, I, III, and aVF indicate disease of the right coronary or left circumflex arteries (surprisingly, the latter two cannot be differentiated by ECG criteria).
Clinical Lead Systems for Detecting Ischemia
Early clinical reports of intraoperative monitoring using the V 5 lead in high-risk patients were based on observations during exercise testing, in which bipolar configurations of V 5 demonstrated high sensitivity for myocardial ischemia detection (up to 90%). Subsequent studies using 12-lead monitoring (torso-mounted for stability during exercise) confirmed the sensitivity of the lateral precordial leads. Some studies, however, reported higher sensitivity for leads V 4 or V 6 compared with V 5 , followed by the inferior leads (in which most false-positive responses were reported).
Intraoperative Lead Systems
The cardiac anesthesiologist encounters a variety of ECG changes consistent with myocardial ischemia or infarction at many phases of the perioperative period in patients undergoing cardiac operations. In the majority of these patients (ie, those with known CAD), the sensitivity and specificity of the major signs described are high, and few false-positive or false-negative changes are encountered. However, the abnormal physiology of CPB, including acute changes in temperature, electrolyte concentrations, and catecholamine levels can significantly influence sensitivity and specificity. In addition, patients undergoing valve replacement, even those without coronary artery lesions, can develop significant subendocardial and transmural ischemia (ie, coronary artery embolus of valve calcification, vegetations, or air).
Detecting and recognizing the clinical significance of various ECG signs of ischemia or infarction and coordinating the findings with transesophageal echocardiography (TEE) can enhance patient care in the acute setting, as in emergency treatment of coronary artery spasm or air embolus, or by alerting the surgeon that myocardial revascularization may have been inadequate. This may lead to reexploration of a saphenous vein or internal mammary artery anastomosis, especially if the TEE data support the diagnosis of ischemia.
The early reports recommending routine intraoperative monitoring of V 5 in high-risk patients cited exercise tolerance tests as the source of their recommendations. Subsequently, the recommended leads for intraoperative monitoring, based on several clinical studies, did not differ substantially from those used during exercise testing, although considerable controversy on the optimal leads persists in both clinical settings. The use of continuous ECG monitoring in the coronary care unit has received increasing attention. A clinical study using continuous, computerized 12-lead ECG analysis in a mixed cohort reported that almost 90% of changes involved ST-segment depression alone (75% in V 5 and 61% in V 4 ). In approximately 70% of patients, significant changes were observed in multiple leads. The sensitivity of each of the 12 leads in that study is shown in Fig. 9.5 . When considered in combination, sensitivity for the standard clinical combination of leads II and V 5 was 80%.
Electrocardiographic Changes With Pacemakers, Respirations, Electrolytes, and Medications
Use of the inferior leads (II, III, aVF) allows superior discrimination of P-wave morphology and facilitates the visual diagnosis of arrhythmias and conduction disorders ( Appendix 9.1 ).With the increasing use of implantable defibrillators and automatic external defibrillators to treat ventricular fibrillation and ventricular tachycardia, considerable interest exists in the refinement of arrhythmia detection algorithms and their validation. As expected, the accuracy of these devices for detecting ventricular arrhythmias is high, but it is much lower for detecting atrial arrhythmias. Detection of pacemaker spikes may be complicated by very low-amplitude signals related to bipolar pacing leads, amplitude varying with respiration, and total body fluid accumulation. Most critical care and ambulatory monitors incorporate pacemaker spike enhancement for small high-frequency signals (typically 5–500 mV with 0.5- to 2-ms pulse duration) to facilitate recognition. However, this can cause artifact if high-frequency noise is present within the lead system.
A promising application of the electrocardiogram is to correlate respiratory variation in wave amplitude with patients’ volume responsiveness. The R wave, especially in lead II (RII), shows consistent respiratory amplitude variation during positive-pressure mechanical ventilation. This variation is likely caused by the “Brody effect,” a theoretical analysis of left ventricular volume and electrical conductance. RII-wave amplitude variation may be used as a dynamic index of volume responsiveness in a mechanically ventilated patient, similar to the use of arterial pulse contour analysis and esophageal Doppler monitoring to derive pulse-pressure and stroke volume variation as dynamic measures of fluid responsiveness. Real-time, intraoperative RII-wave amplitude variation has the potential to become a truly noninvasive monitor for fluid responsiveness; however, at present, no commercially available intraoperative monitoring systems provide ECG R-wave amplitude variation measures.
Electrocardiographic Changes Resulting From Electrolyte Disorders
Cardiac myocytes exhibit a long action potential (200–400 ms) compared with neurons and skeletal muscle (1–5 ms). Multiple different channels are involved in cardiac muscle depolarization and repolarization. Sodium and calcium channels are the primary carriers of depolarizing current in both atria and ventricles. Inactivation of these currents and activation of potassium channels are predominantly involved in repolarizing the cardiac cells, thereby reestablishing the negative resting membrane potential. Thus it is not surprising that perturbations in the plasma concentrations of potassium and calcium ions lead to changes in the finely tuned cardiac electrical activity and the surface electrocardiogram. They typically cause changes in repolarization (ST-T-U waves) and can also lead to QRS complex prolongation.
Hyperkalemia
Hyperkalemia is not an uncommon occurrence in patients undergoing cardiac surgical procedures with CPB. Hyperkalemia affects repolarization of cardiac cells. Although progressive changes in the surface electrocardiogram with increasing levels of potassium have been described, the correlation between serum potassium levels and ECG changes is not strong. Typically, ECG changes start with narrowing and peaking of the T waves. Further elevation of extracellular potassium leads to prolongation of the QRS complex. The reason is delayed AV conduction, and an AV block may appear. These changes are typically followed by prolongation of the PR interval, flattening of the P waves, and loss of the P wave because the high potassium levels delay the spread of the cardiac activating impulse through the myocardium. Further increase in plasma potassium levels cause sine waves, which can progress to asystole or ventricular fibrillation. Hyperkalemia may also reduce the myocardial response to artificial pacemaker stimulation. These changes are all seen with use of potassium cardioplegia.
Hypokalemia
Because potassium channels and ions are significantly involved in cardiac repolarization, it is not surprising that hypokalemia prolongs ventricular repolarization. This results in characteristic reversal in the relative amplitudes of the T and U waves. T-wave flattening or inversion is noted, whereas U waves become more prominent. The U-wave prominence is caused by the prolongation of the recovery phase of the cardiac action potential. This can lead to the life-threatening torsades de pointes type of ventricular arrhythmia. Slight depression of the ST segment may also occur, as well as increased amplitude and width of the P waves with prolongation of the PR interval.
Hypocalcemia and Hypercalcemia
The ventricular recovery time, as represented on the electrocardiogram by the QTc interval, is altered by the extremes of serum calcium. Hypocalcemia can cause a prolonged QTc interval (ST portion), whereas hypercalcemia shortens the QTc interval. In hypocalcemia, the prolonged QT interval may be accompanied by terminal T-wave inversions. In extreme hypercalcemia, an increase in QRS complex, biphasic T waves, and Osborn waves has been described.
Medications
Many antiarrhythmic medications are used in the perioperative period in patients undergoing cardiac surgical procedures. Generally, drugs that increase the duration of the cardiac action potential prolong the QT interval. These include class Ia and Ic antiarrhythmic drugs (eg, quinidine, procainamide), phenothiazines, antidepressants, haloperidol, and atypical antipsychotic agents. Intravenous amiodarone, commonly used in the management of perioperative arrhythmias, also causes QT-interval prolongation. Other class III antiarrhythmic drugs (eg, sotalol) cause QT-interval prolongation as well. Unlike class Ia and III antiarrhythmic drugs, digitalis glycosides shorten the QT interval and often cause “scooping” of the ST-T wave complex.
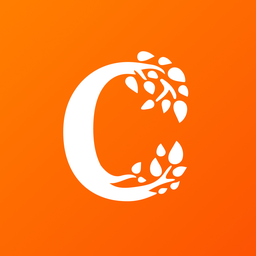
Full access? Get Clinical Tree
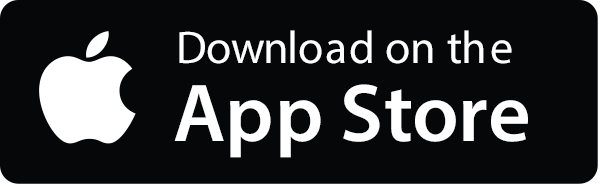
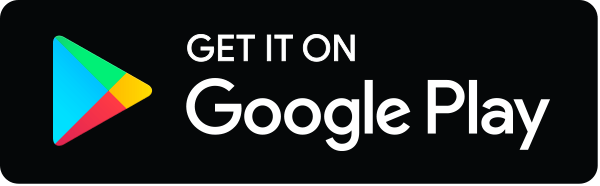