Electrical Nerve Stimulators and Localization of Peripheral Nerves
Martin Simpel and Andre van Zundert
History of Electrical Nerve Stimulation
Quick Facts
• 1780: Galvani1 was the first to describe the effect of electrical neuromuscular stimulation
• 1912: Perthes2 developed and described an electrical nerve stimulator
• 1955: Pearson3 introduced the concept of insulated needles for nerve location
• 1962: Greenblatt and Denson4 introduced a portable solid-state nerve stimulator with variable current output and described its use for nerve location
• 1973: Montgomery et al5 demonstrated that noninsulated needles require significantly higher current amplitudes than the insulated needles
• 1984: Ford et al6 reported a lack of accuracy with noninsulated needles once the needle tip passed the target nerve
• Ford et al suggested the use of nerve stimulators with a constant current source, based on the comparison of the electrical characteristics of peripheral nerve stimulators7,8
The use of nerve stimulation became commonplace in clinical practice only in the mid- to late 1990s. Research on the needle–nerve relationship and the effect of stimulus duration ensued.9–11 More recently, the principles of electrical nerve stimulation were applied to surface mapping of peripheral nerves using percutaneous electrode guidance (PEG)12–15 for confirmation and epidural catheter placement16–18 and peripheral catheter placement.19 This chapter discusses the electrophysiology of nerve stimulation, electrical nerve stimulators, various modes of localization of peripheral nerves, and integration of the technology into the realm of modern regional anesthesia.
What Is Peripheral Electrical Nerve Stimulation?
Nerve stimulation is a commonly used method for localizing nerves before the injection of local anesthetic. Electrical nerve stimulation in regional anesthesia is a method of using a low-intensity (up to 5 mA) and short-duration (0.05–1 ms) electrical stimulus (at 1–2 Hz repetition rate) to obtain a defined response (muscle twitch or sensation) to locate a peripheral nerve or nerve plexus with an (insulated) needle. The goal is to inject a certain amount of local anesthetic in close proximity to the nerve to block nerve conduction and provide a sensory and motor block for surgery and/or, eventually, analgesia for pain management. The use of nerve stimulation can also help to avoid an intraneural intrafascicular injection and, consequently, nerve injury.
Electrical nerve stimulation can be used for a single-injection technique, as well as for guidance during the insertion of continuous nerve block catheters. More recently, ultrasound (US) guidance and, in particular, the so-called dual guidance technique in which both techniques (peripheral nerve stimulation [PNS] and US) are combined, has become a common practice in many institutions.
Indications for the Use of PNS
In principle, almost all plexuses or other larger peripheral nerves can be located using PNS.20 The goal of nerve stimulation is to place the tip of the needle (more specifically, its orifice for injection) in close proximity to the target nerve to inject the local anesthetic in the vicinity of the nerve. The motor response (twitch) to PNS is objective and reliable and independent from the patient’s (subjective) response. Nerve stimulation is often helpful to confirm that the structure imaged with ultrasound (US) is actually the nerve that is sought. This is because the needle–nerve relationship may not always be visualized on US; an unexpected motor response can occur, alerting the operator that the needle tip is already in close proximity to the nerve. Likewise, the occurrence of a motor response at a current intensity of <0.3-0.2 mA can serve as an indicator of an intraneural needle placement. Although this response may not always be present even with an intraneural needle position (low sensitivity), its presence is always indicative of intraneural placement (high specificity).
The disadvantages of PNS are the need for additional equipment (nerve stimulator and insulated needles), the greater cost of insulated needles, and abnormal physiology or anatomy where it may be difficult to elicit a motor response.
Basics of Neurophysiology and Electrophysiology
Membrane Potential, Resting Potential, Depolarization, Action Potential, and Impulse Propagation
All living cells have a membrane potential (a voltage potential across their membrane, measured from the outside to the inside), which varies (depending on the species and the cell type) from about −60 mV to −100 mV. Nerve and muscle cells in mammals typically have a membrane potential (resting potential) of about −90 mV.
Only nerve and muscle cells have the capability of producing uniform electrical pulses, the so-called action potentials (also called spikes), which are propagated along their membranes, especially along the long extensions of nerve cells (nerve fibers, axons). A decrease in the electric potential difference (e.g., from −90 mV to −55 mV, or depolarization) elicits an action potential. If the depolarization exceeds a certain threshold, an action potential or a series of action potentials is generated by the nerve membrane (also called firing) according to the all-or-nothing rule, resulting in propagation of the action potential along the nerve fiber (axon). To depolarize the nerve membrane from outside the cell (extracellular stimulation), the negative polarity of the electrical stimulus is more effective in removing the positive charge from the outside of the membrane. This in turn decreases the potential across the membrane toward the threshold level.
There are several types of nerve fibers. Each fiber type can be distinguished anatomically by their diameter and degree of myelinization. Myelinization is formed by an insulating layer of Schwann cells wrapped around the nerve fibers. These characteristics largely determine the electrophysiologic behavior of different nerve fibers, that is, the speed of impulse propagation of action potentials and the threshold of excitability. Most commonly, the distinguishing features are motor fibers (e.g., Aα, Aβ) and pain fibers (C). The Aα motor fibers have the largest diameter and highest degree of myelinization and therefore the highest speed of impulse propagation and a relatively low threshold level to external stimulation. C-fibers (which transmit severe, dull pain) have very little to no myelinization and are of smaller diameter. Consequently, the speed of propagation in these fibers is relatively low, and the threshold levels to external stimulation, in general, are higher.
There are several other, efferent fibers, which transmit responses from various skin receptors or muscle spindles (Aδ). These are thinner than Aα fibers and have less myelinization. Some of these (afferent) sensory fibers, having a relatively low threshold level, transmit the typical tingling sensation associated with a lower level of pain sensation when electrically stimulated. Such sensation can occur during transcutaneous stimulation before a motor response is elicited.
The basic anatomic structure of myelinated Aα fibers (motor) and nonmyelinated C fibers (pain) is shown schematically in Figure 4-1. The relationship between different stimuli and the triggering of the action potential in motor and pain fibers is illustrated in Figures 4-2A, B.
FIGURE 4-1. (A) Schematic anatomic and electrophysiologic structure of nerve fibers of myelinated and (B) unmyelinated nerve fibers.
FIGURE 4-2. (A) Action potential, threshold level, and stimulus. Motor fibers have a short chronaxy because of the relatively low capacitance of their myelinated membrane (only the area of the nodes of Ranvier count; see Figure 4-1), therefore, it takes only a short time to depolarize the membrane up to the threshold level. (B) Pain fibers have a long chronaxy because of the higher capacitance of their nonmyelinated membrane (the entire area of the membrane counts); therefore, it takes a longer time to depolarize the membrane up to the threshold level. Short impulses (as indicated by the vertical dotted line) would not be able to depolarize the membrane to its threshold level.
Threshold Level, Rheobase, Chronaxy
A certain minimum current intensity is necessary at a given pulse duration to reach the threshold level of excitation. The lowest threshold current (at infinitely long pulse durations) is called rheobase. The pulse duration (pulse width) at double the rheobase current is called chronaxy. Electrical pulses with the duration of the chronaxy are most effective (at relatively low amplitudes) to elicit action potentials. This is the reason why motor response can be elicited at such short pulse duration (e.g., 0.1 ms) at relatively low current amplitudes while avoiding the stimulation of C-type pain fibers. Typical chronaxy figures are 50 to 100 μs (Aα fibers), 170 μs (AΔ fibers), and ≥400 μs (C fibers). Figure 4-3 illustrates the relationship of the rheobase to chronaxy for motor fibers versus pain nerve fibers.
FIGURE 4-3. (A) Comparison of threshold curves, chronaxy, and rheobase level of motor (high speed) and pain fibers (low speed). (B) Experimental data, threshold amplitudes obtained with percutaneous stimulation (Stimuplex Pen and Stimuplex HNS 12). Stimulation obtained with percutaneous stimulation of the median nerve near the wrist looking for motor response of the thumb. (C) Experimental data, threshold amplitudes obtained with percutaneous stimulation (Stimuplex Pen and Stimuplex HNS 12). Stimulation of the median and radial nerves near the wrist and at the midforearm looking for electric paresthesia (tingling sensation) in the middle and ring finger (median nerve) or superficial pain sensation near the wrist (radial nerve), respectively.
Impedance, Impulse Duration, and Constant Current
The electrical circuit is formed by the nerve stimulator, the nerve block needle and its tip, the tissue characteristics of the patient, the skin, the skin electrode (grounding electrode), and the cables connecting all the elements. The resistance of this circuit is not just a simple Ohm’s resistor equation because of the specific capacitances of the tissue, the electrocardiogram (ECG) electrode to skin interface, and the needle tip, which influence the overall resistance. The capacitance in the described circuit varies with the frequency content of the stimulation current, and it is called impedance, or a so-called complex resistance, which depends on the frequency content of the stimulus. In general, the shorter the impulse, the higher its frequency content, and, consequently, the lower the impedance of a circuit with a given capacitance. Conversely, a longer pulse duration has a lower frequency content. As an example, for a 0.1-ms stimulus, the main frequency content is 10 kHz plus its harmonics; whereas for a 1.0-ms impulse, the main frequency content is 1 kHz plus harmonics. In reality, the impedance of the needle tip and the electrode to skin impedance have the highest impact. The impedance of the needle tip largely depends on the geometry and insulation (conductive area). The electrode to skin impedance can vary considerably between individuals (e.g., type of skin, hydration status) and can be influenced by the quality of the ECG electrode used.
Because of the variable impedance in the circuit, created primarily by the needle tip and electrode to skin interface, a nerve stimulator with a constant current source and sufficient (voltage) output power is important to use to compensate for the wide range of impedances encountered clinically.
Clinical Use of PNS
Proper Setup and Check of the Equipment
The following are a few important aspects for successful electrolocalization of the peripheral nerves using PNS:
• Use a high-quality nerve stimulator and a high-accuracy constant current source.
• Use insulated nerve stimulation needles with a small conductive area at the tip. The smaller the conductive area, the higher the current density is at the tip, and the greater spatial discrimination in the near field.
• Use high-quality skin electrodes with a low impedance.
• Before starting the procedure, check for the proper functioning of the nerve stimulator and the connecting cables.
• During nerve stimulator-assisted nerve localization, the negative pole (cathode) should be connected to the stimulating electrode (needle) and the positive pole (anode) to the patient’s skin.
• The design of the connectors should prevent a faulty polarity connection.
• Connect the nerve stimulation needle to the nerve stimulator (which should be turned on), and set the current amplitude and duration to the desired levels.
For superficial blocks, select 1.0 mA as a starting current intensity.
For deep blocks, select 1.5 mA as a starting current intensity.
Select between 0.1 and 0.3 ms of current duration for most purposes.
• For more technical details and how to operate a specific nerve stimulator, refer to the instructions for use supplied with the stimulator.
Transcutaneous Nerve Mapping
Electrolocalization of peripheral nerves is typically accomplished by inserting a needle into the tissue and advancing the needle toward the expected location of the nerve(s) of interest. However, a nerve mapping pen can be used to locate superficial nerves (up to a maximum depth of approximately 3 cm) with transcutaneous nerve stimulation before the nerve block needle is inserted. Transcutaneous nerve mapping is particularly useful when identifying the best site for needle insertion in patients with difficult anatomy or when the landmarks prove difficult to indentify. Figure 4-4 shows three examples of commercially available nerve mapping pens.
FIGURE 4-4
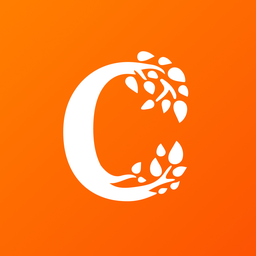
Full access? Get Clinical Tree
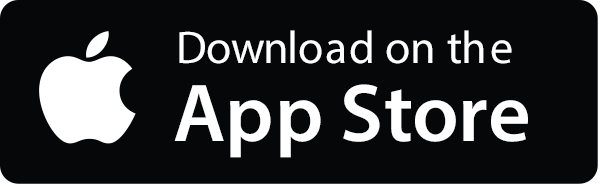
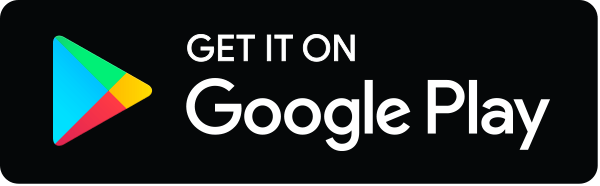