Introduction
The major goals in neurosurgical anesthesia are to provide adequate tissue perfusion to the brain (and spinal cord) so that the regional metabolic demand is met and to provide adequate surgical conditions (a “relaxed brain”). If anesthetic drugs or anesthetic techniques are improperly used, they can worsen the existing intracranial pathologic condition and may produce new damage. Some anesthetics or anesthetic techniques may help protect the brain subjected to metabolic stress or even ameliorate damage from such an insult. Thus, knowledge of the effects of anesthetics and anesthetic techniques on cerebral circulation, metabolism, and intracranial pressure (ICP) both in normal and pathologic conditions is important. In addition, special attention must be paid in the case of functional neurosurgery or minimally invasive surgery, such as awake surgery, stereotaxic surgery, identification of epileptic foci, and neuroradiological interventional procedures, in which anesthesiologists should consider using anesthetics and adjuvant drugs that allow control of asleep-awake-asleep status or sedation with analgesia and no or minimal interference with brain electrophysiologic monitoring or neurologic findings. While providing such a state, the anesthesiologist should ensure patency of the airway with well-maintained ventilation and circulatory stability.
In this chapter, physiologic and pharmacologic considerations in relation to neurosurgical anesthesia are summarized, followed by a review of the effects of anesthetics and other drugs on cerebral blood flow (CBF), cerebral metabolism, and ICP, focusing on human data. Data from animal studies are cited only when there are not sufficient human data. The clinical relevance of these issues to the practice of neurosurgical anesthesia is discussed.
Physiologic and pharmacologic considerations in relation to neurosurgical anesthesia
Blood Flow and Metabolism Changes in Relation to Functional Changes
Under physiologic conditions, the brain vessel diameter changes within seconds in response to the changes in neuronal activity that immediately influence metabolic demand. Although the cellular mechanisms underlying the coupling of neuronal activation to cerebral blood vessel responses are not fully determined, it has been proposed that the energy (metabolic) demand caused by neuronal activity increases blood flow. The underlying metabolic signals could be a lack of O 2 or glucose, or the production of CO 2 . However, studies have demonstrated that blocking the enzymes that generate nitric oxide (NO) and arachidonic acid derivatives downstream of glutamate receptors greatly reduces functional hyperemia with little effect on the energy use associated with neuronal activity. , From these data, a “feed-forward mechanism” has been proposed in which glutamate released from presynaptic nerve terminals activates both neurons and astrocytes, leading to the release of vasoactive substances from both cell types. As this feed-forward mechanism is not driven by energy demand, the fractional increase in blood flow induced by sustained neuronal activity is greater than the increase in neuronal adenosine triphosphate (ATP) consumption. The vasoactive substances include NO, prostaglandin E 2 (PGE 2 ), potassium ions (K + ), epoxyeicosatrienoic acid (EET), and arachidonic acid. NO, PGE 2 , K + , and EET dilate vessels. In contrast, arachidonic acid released from astrocytes is converted to 20-hydroxy- eicosatetraenoic acid (20-HETE) in vascular smooth muscle cells, which constricts vessels. Whether astrocytic activation leads to vasodilation or vasoconstriction may depend on preexisting vessel tone.
Traditionally, blood flow is controlled solely by arteriole smooth muscle. However, recent evidence suggests that vasodilatory substances, such as PGE 2 or related substances, can actively relax the pericytes that regulate the diameter of capillaries. In an animal study, it was demonstrated that capillaries dilate before arterioles in response to neuronal activity and that most of the increase in blood flow can be attributed to capillary dilation. The role of pericytes in controlling cerebral blood flow remains to be determined.
As neurons have a limited energy reserve, sufficient ATP should be generated to match energy demand, which changes dramatically with neuronal activity. To explain how ATP is generated on demand, the “astrocyte-neuron lactate shuttle hypothesis” was proposed, in which astrocytic activation by glutamate released from neurons stimulates glucose uptake into astrocytes; glucose is processed glycolytically, resulting in a release of lactate as an energy substrate for neurons. This hypothesis is based on the finding that cerebral activation resulted in a much greater increase in glucose consumption than in oxygen consumption. However, a smaller discrepancy between the increase of glucose and oxygen use was also reported. Recent quantitative work has shown that most of the ATP produced in response to increased neuronal activity is generated by oxidative phosphorylation. It appears that the extent to which astrocytes feed neurons still remains controversial.
Anesthetics cause functional alterations in the central nervous system and produce metabolic changes. In general, intravenous anesthetics decrease cerebral metabolic rate (CMR) and CBF in parallel fashion, whereas most inhalational anesthetics decrease CMR with an increase in CBF. At first sight, the coupling of CMR and CBF is maintained with intravenous anesthetics, whereas it is lost with inhalational anesthetics. However, a strong correlation exists between CMR and CBF within individual brain structures during anesthesia. Indeed, during burst and suppression phases of electroencephalogram (EEG) with isoflurane anesthesia, cerebral blood flow velocity of middle cerebral artery (Vmca) appears to increase and decrease, respectively. , In addition, seizure activity or noxious stimuli during anesthesia produce parallel increases in CBF and CMR. Because the net effect of anesthetics on CBF is a balance between their direct effects on cerebral vessels and indirect effects caused by CMR changes, it is probable that the coupling of CMR and CBF is maintained with anesthetics but is modified by direct effects of anesthetics on vascular tone.
Blood Flow Changes in Relation to Cerebral Perfusion Pressure and CO 2
Cerebral perfusion pressure (CPP) and carbon dioxide tension in the arterial blood (PaCO 2 ) are the important variables that influence CBF. Autoregulation is the physiologic maintenance of constant CBF over a wide range of CPP values. Traditionally, CPP is determined as the difference between mean arterial blood pressure (MABP) and the greater of ICP or CVP. In the patient with intracranial hypertension, effective downstream pressure is determined by ICP. Advances in flow measurement techniques have demonstrated beat-to-beat flow changes and the concept of apparent zero flow pressure, at which flow ceases. Apparent zero flow pressure has been proposed as an estimate of critical closing pressure that may better estimate CPP. Zero-flow pressure is extrapolated by linear regression analysis of the arterial blood-pressure–Vmca relationship. The arterial blood pressure-axis intercept of the regression line determines zero-flow pressure. In conditions of increased cerebrovascular tone, such as hypocapnia or pharmacologically induced vasoconstriction, ICP does not uniquely determine effective downstream pressure.
CO 2 can produce marked changes in cerebrovascular resistance (CVR) and CBF. Over a range of PaCO 2 values of 20 to 80 mmHg, for each 1 mmHg increase or decrease in PaCO 2 there is a 2% to 4% increase or decrease in CBF. Changes in the extracellular hydrogen ion (H + ) concentration, NO, prostanoids, cyclic nucleotides, intracellular calcium, and potassium channel activity have been regarded as regulatory factors for cerebrovascular reactivity to CO 2 . Compared with adults, children have less cerebral reactivity to CO 2 changes. Whether this difference is related to possible domination of prostaglandin and cyclic guanosine monophosphate in regulating vascular tone in children remains to be determined.
As CO 2 affects CVR and CBF, the autoregulation curve changes according to CO 2 levels. During hypercapnia, the plateau ascends and shortens, the lower limit shifts rightward, and the upper limit shifts leftward. In contrast, during hypocapnia, the plateau descends and the lower limit remains unchanged. How the upper limit moves during hypocapnia is not clear.
Changes in Cerebral Blood Flow and Intracranial Pressure Regulation in Pathologic Conditions
Patients who undergo neurosurgery may have various types of intracranial pathologic conditions as well as systemic diseases, and their responses to anesthetics may be different from those of normal subjects. Brain tissue hypoxia, acidosis, and edema are the main pathologic consequences of most brain disorders. Cerebral vasoparalysis occurs, and coupling between blood flow and metabolism is impaired. Under these circumstances, autoregulation and CO 2 reactivity are also disturbed. Strict blood pressure control and respiratory management are required.
In the event of focal cerebral ischemia, hypercapnia can dilate the vessels in the normal area but not in the damaged area, and, consequently, blood flow may be shunted from the ischemic to the normal area (intracerebral steal or the “reversed Robin Hood effect”). As intracerebral steal has been documented in acute ischemic stroke patients, hypercapnia should be avoided in these patients. Conversely, hypocapnia can divert blood from the normal area to the ischemic area (inverse intracerebral steal, or the “Robin Hood effect”). However, no beneficial effect was found in an animal experiment when hyperventilation was initiated at 1 hour after focal cerebral ischemia. Because of a lack of evidence indicating the possible beneficial effect on outcome, hyperventilation cannot be recommended in patients who have experienced stroke.
Although the effect is not confirmed for every anesthetic, experimental data in animals suggest that intracerebral steal or inverse intracerebral steal may also be induced pharmacologically. However, the effect of anesthetics on cerebral blood flow redistribution is unpredictable because anesthetics modulate cerebral vessel diameter by both their direct vasoactive property and indirect effects caused by CMR changes.
Anesthesia alters ICP through changes in cerebral blood volume (CBV). Although correlation between CBV and CBF does not always exist, the changes in CBV, in general, appear to be proportional to the changes in CBF. Therefore, an increase in CBF causes an increase in CBV and, thus ICP. Increases in blood pressure, especially when autoregulation is impaired, also produce an increase in CBV. Mechanical effects, such as the patient’s position and respiratory pattern (by influencing intrathoracic pressure) also may affect ICP. , Muscle activity during the patient’s movement may raise central venous pressure (CVP) and ICP. Anesthetic agents also affect ICP by changing the rate of production and reabsorption of cerebrospinal fluid (CSF) (see Chapter 3 ). Intracranial physiology and pathophysiology in relation to the use of anesthetics and adjuvant drugs are summarized in Fig. 4.1 .
Physiologic and pharmacologic considerations in relation to neurosurgical anesthesia
Blood Flow and Metabolism Changes in Relation to Functional Changes
Under physiologic conditions, the brain vessel diameter changes within seconds in response to the changes in neuronal activity that immediately influence metabolic demand. Although the cellular mechanisms underlying the coupling of neuronal activation to cerebral blood vessel responses are not fully determined, it has been proposed that the energy (metabolic) demand caused by neuronal activity increases blood flow. The underlying metabolic signals could be a lack of O 2 or glucose, or the production of CO 2 . However, studies have demonstrated that blocking the enzymes that generate nitric oxide (NO) and arachidonic acid derivatives downstream of glutamate receptors greatly reduces functional hyperemia with little effect on the energy use associated with neuronal activity. , From these data, a “feed-forward mechanism” has been proposed in which glutamate released from presynaptic nerve terminals activates both neurons and astrocytes, leading to the release of vasoactive substances from both cell types. As this feed-forward mechanism is not driven by energy demand, the fractional increase in blood flow induced by sustained neuronal activity is greater than the increase in neuronal adenosine triphosphate (ATP) consumption. The vasoactive substances include NO, prostaglandin E 2 (PGE 2 ), potassium ions (K + ), epoxyeicosatrienoic acid (EET), and arachidonic acid. NO, PGE 2 , K + , and EET dilate vessels. In contrast, arachidonic acid released from astrocytes is converted to 20-hydroxy- eicosatetraenoic acid (20-HETE) in vascular smooth muscle cells, which constricts vessels. Whether astrocytic activation leads to vasodilation or vasoconstriction may depend on preexisting vessel tone.
Traditionally, blood flow is controlled solely by arteriole smooth muscle. However, recent evidence suggests that vasodilatory substances, such as PGE 2 or related substances, can actively relax the pericytes that regulate the diameter of capillaries. In an animal study, it was demonstrated that capillaries dilate before arterioles in response to neuronal activity and that most of the increase in blood flow can be attributed to capillary dilation. The role of pericytes in controlling cerebral blood flow remains to be determined.
As neurons have a limited energy reserve, sufficient ATP should be generated to match energy demand, which changes dramatically with neuronal activity. To explain how ATP is generated on demand, the “astrocyte-neuron lactate shuttle hypothesis” was proposed, in which astrocytic activation by glutamate released from neurons stimulates glucose uptake into astrocytes; glucose is processed glycolytically, resulting in a release of lactate as an energy substrate for neurons. This hypothesis is based on the finding that cerebral activation resulted in a much greater increase in glucose consumption than in oxygen consumption. However, a smaller discrepancy between the increase of glucose and oxygen use was also reported. Recent quantitative work has shown that most of the ATP produced in response to increased neuronal activity is generated by oxidative phosphorylation. It appears that the extent to which astrocytes feed neurons still remains controversial.
Anesthetics cause functional alterations in the central nervous system and produce metabolic changes. In general, intravenous anesthetics decrease cerebral metabolic rate (CMR) and CBF in parallel fashion, whereas most inhalational anesthetics decrease CMR with an increase in CBF. At first sight, the coupling of CMR and CBF is maintained with intravenous anesthetics, whereas it is lost with inhalational anesthetics. However, a strong correlation exists between CMR and CBF within individual brain structures during anesthesia. Indeed, during burst and suppression phases of electroencephalogram (EEG) with isoflurane anesthesia, cerebral blood flow velocity of middle cerebral artery (Vmca) appears to increase and decrease, respectively. , In addition, seizure activity or noxious stimuli during anesthesia produce parallel increases in CBF and CMR. Because the net effect of anesthetics on CBF is a balance between their direct effects on cerebral vessels and indirect effects caused by CMR changes, it is probable that the coupling of CMR and CBF is maintained with anesthetics but is modified by direct effects of anesthetics on vascular tone.
Blood Flow Changes in Relation to Cerebral Perfusion Pressure and CO 2
Cerebral perfusion pressure (CPP) and carbon dioxide tension in the arterial blood (PaCO 2 ) are the important variables that influence CBF. Autoregulation is the physiologic maintenance of constant CBF over a wide range of CPP values. Traditionally, CPP is determined as the difference between mean arterial blood pressure (MABP) and the greater of ICP or CVP. In the patient with intracranial hypertension, effective downstream pressure is determined by ICP. Advances in flow measurement techniques have demonstrated beat-to-beat flow changes and the concept of apparent zero flow pressure, at which flow ceases. Apparent zero flow pressure has been proposed as an estimate of critical closing pressure that may better estimate CPP. Zero-flow pressure is extrapolated by linear regression analysis of the arterial blood-pressure–Vmca relationship. The arterial blood pressure-axis intercept of the regression line determines zero-flow pressure. In conditions of increased cerebrovascular tone, such as hypocapnia or pharmacologically induced vasoconstriction, ICP does not uniquely determine effective downstream pressure.
CO 2 can produce marked changes in cerebrovascular resistance (CVR) and CBF. Over a range of PaCO 2 values of 20 to 80 mmHg, for each 1 mmHg increase or decrease in PaCO 2 there is a 2% to 4% increase or decrease in CBF. Changes in the extracellular hydrogen ion (H + ) concentration, NO, prostanoids, cyclic nucleotides, intracellular calcium, and potassium channel activity have been regarded as regulatory factors for cerebrovascular reactivity to CO 2 . Compared with adults, children have less cerebral reactivity to CO 2 changes. Whether this difference is related to possible domination of prostaglandin and cyclic guanosine monophosphate in regulating vascular tone in children remains to be determined.
As CO 2 affects CVR and CBF, the autoregulation curve changes according to CO 2 levels. During hypercapnia, the plateau ascends and shortens, the lower limit shifts rightward, and the upper limit shifts leftward. In contrast, during hypocapnia, the plateau descends and the lower limit remains unchanged. How the upper limit moves during hypocapnia is not clear.
Changes in Cerebral Blood Flow and Intracranial Pressure Regulation in Pathologic Conditions
Patients who undergo neurosurgery may have various types of intracranial pathologic conditions as well as systemic diseases, and their responses to anesthetics may be different from those of normal subjects. Brain tissue hypoxia, acidosis, and edema are the main pathologic consequences of most brain disorders. Cerebral vasoparalysis occurs, and coupling between blood flow and metabolism is impaired. Under these circumstances, autoregulation and CO 2 reactivity are also disturbed. Strict blood pressure control and respiratory management are required.
In the event of focal cerebral ischemia, hypercapnia can dilate the vessels in the normal area but not in the damaged area, and, consequently, blood flow may be shunted from the ischemic to the normal area (intracerebral steal or the “reversed Robin Hood effect”). As intracerebral steal has been documented in acute ischemic stroke patients, hypercapnia should be avoided in these patients. Conversely, hypocapnia can divert blood from the normal area to the ischemic area (inverse intracerebral steal, or the “Robin Hood effect”). However, no beneficial effect was found in an animal experiment when hyperventilation was initiated at 1 hour after focal cerebral ischemia. Because of a lack of evidence indicating the possible beneficial effect on outcome, hyperventilation cannot be recommended in patients who have experienced stroke.
Although the effect is not confirmed for every anesthetic, experimental data in animals suggest that intracerebral steal or inverse intracerebral steal may also be induced pharmacologically. However, the effect of anesthetics on cerebral blood flow redistribution is unpredictable because anesthetics modulate cerebral vessel diameter by both their direct vasoactive property and indirect effects caused by CMR changes.
Anesthesia alters ICP through changes in cerebral blood volume (CBV). Although correlation between CBV and CBF does not always exist, the changes in CBV, in general, appear to be proportional to the changes in CBF. Therefore, an increase in CBF causes an increase in CBV and, thus ICP. Increases in blood pressure, especially when autoregulation is impaired, also produce an increase in CBV. Mechanical effects, such as the patient’s position and respiratory pattern (by influencing intrathoracic pressure) also may affect ICP. , Muscle activity during the patient’s movement may raise central venous pressure (CVP) and ICP. Anesthetic agents also affect ICP by changing the rate of production and reabsorption of cerebrospinal fluid (CSF) (see Chapter 3 ). Intracranial physiology and pathophysiology in relation to the use of anesthetics and adjuvant drugs are summarized in Fig. 4.1 .
Effects of specific anesthetic drugs and other drugs
Inhalational Anesthetics
In general, all inhalational anesthetics are cerebral vasodilators and possess the capability of increasing ICP. Inhalational anesthetics, with the possible exception of nitrous oxide (N 2 O), usually depress metabolism. Although the coupling of neuronal activation to cerebral blood vessel responses seems to work even with high concentrations of inhalational anesthetics, direct vasodilation surpasses indirect vasoconstriction by the reduction of CMRO 2 , resulting in a higher CBF/CMRO 2 ratio. Table 4.1 summarizes the effects of inhalational anesthetics on CBF, CMR, and ICP.
Cerebral Blood Flow | Cerebral Metabolic Rate | Intracranial Pressure | |
---|---|---|---|
N 2 O | ↑↑ | ↑ or → | ↑↑ |
Xenon | ↓ (Gray) ↑ (White) | ↓ | ↑ or → |
Isoflurane | ↑ or → | ↓↓ | → or ↗ or ↑ |
Sevoflurane | ↓ or → or ↗ | ↓ or ↓↓ | → or ↗ or ↑ |
Desflurane | ↓ or ↑ | ↓↓ | ↑ or → |
Nitrous Oxide
It is generally agreed that N 2 O increases CBF, CMR, and ICP; however, in humans, some studies did not observe an increase in CMR. The magnitude of changes varies substantially. The cause of this variation may be the concentrations examined and its use in combination with other drugs that may modify its original effect. The most dramatic increases in CBF and ICP occurred when N 2 O was administered alone or with minimal background anesthetics. The increases in CBF and CMRO 2 with N 2 O do not appear to be related solely to the sympathetic hyperactivity. N 2 O appears to have no direct vasodilating effect.
Marked heterogeneity in regional cerebral blood flow (rCBF), regional CBV (rCBV), and regional CMR (rCMR) during administration of N 2 O alone has been revealed with positron emission tomography (PET) and magnetic resonance imaging (MRI). Subanesthetic concentrations of N 2 O (20%) increase rCBF and rCMR in the anterior cingulate cortex, with opposite effects occurring in the posterior cingulate, hippocampus, parahippocampal gyrus, and visual cortices. N 2 O 30% increased CBF in the global gray matter by 22%, with no change detected in global CMRO 2 . At N 2 O 50%, rCBF and rCBV increased in all gray-matter regions, although the increase in rCBF was less pronounced in basal ganglia. Global cerebral metabolic rates for glucose (CMRg) was unchanged with N 2 O 50%, but regional metabolism was changed; regional CMRg increased in the basal ganglia and thalamus and this effect was present 1 hour after discontinuation of N 2 O.
N 2 O, when added to volatile anesthetics, raises CBF, but CMR is either increased or unchanged. Indirect evidence—measurements of Vmca—has shown that N 2 O raises CBF. , In patients with brain tumors, N 2 O increased Vmca, but the increase was completely reversed by hyperventilation. ,
The addition of N 2 O 70% to anesthesia with propofol (EEG isoelectric) in non-neurosurgical patients produced a 20% increase in Vmca with greater oxygen and glucose use in association with EEG activation. A PET study in humans showed that N 2 O 70% counteracted almost all rCBF reductions and some of rCMRO 2 reductions produced by propofol at a dose of clinical anesthesia (EEG activity remained). In contrast, in animal studies, a high dose of thiamylal or pentobarbital has been shown to abolish the N 2 O-induced increases in CBF or CMRO 2 . Regional metabolic studies in rats demonstrated that N 2 O 67% did not change local cerebral metabolic rates for glucose (lCMR g ), with a nearly isoelectric EEG by pentobarbital. Whether the differences in modification of N 2 O-induced increases in CBF or CMR by other anesthetics are due to the differences in species, methods, or the dose ranges of the anesthetics examined is not clear.
An increase in ICP caused by N 2 O has been repeatedly demonstrated. The rise in ICP can be attenuated by prior administration of thiopental, diazepam, or morphine, or by induction of hypocapnia. It is advisable to use hypocapnia, cerebral vasoconstricting drugs, or both, when N 2 O is administered, especially in patients with reduced intracranial compliance.
Some authorities have proposed that N 2 O has neurotoxic properties based on data from experimental animals. It has been reported that N -methyl- d -aspartate (NMDA) receptor blockade during synaptogenesis in the immature brain can induce neuronal degeneration. This effect occurs not only with anesthetics with an NMDA receptor blocking property (N 2 O, xenon, and ketamine) but also with those acting as gamma- aminobutyric acid (GABA) receptor modulators (propofol, midazolam, barbiturates, and isoflurane). However, it has been demonstrated that NMDA receptor antagonists protect against ischemic brain injuries. N 2 O may have both neuroprotective and neurotoxic properties. In humans, post-hoc analysis of data acquired as part of the Intraoperative Hypothermia for Aneurysm Surgery Trial (IHAST) showed no detrimental effect on the long-term gross neurological or neuropsychological outcome with the use of N 2 O during cerebral aneurysm clipping. ,
N 2 O enlarges the volume of potential air space, and thus its use is restricted in patients with intracranial or intravascular air compartment. Further, the incidence of nausea and vomiting appears to increase in patients exposed to N 2 O for more than 1 hour, which may also restrict its use in neurosurgical patients. Since drugs that provide easily controllable analgesia are available, such as remifentanil, the use of N 2 O in neurosurgical anesthesia has decreased.
Xenon
PET showed that xenon 1 MAC decreases absolute rCBF by 11% in the gray matter and increases it by 22% in the white matter, with greater reductions in the cerebellum (by 35%), thalamus (by 23%), and cortical areas (by 9%). The decreased rCBF in the gray matter may be a result of reduced metabolism, as evidenced by the comparable reductions in CMRg in the corresponding brain areas. A later study that determined concomitant changes in rCBF and rCMRg in the same individuals during 1 MAC xenon anesthesia supported the results of these studies. Xenon anesthesia induces a uniform reduction in rCMRg, whereas rCBF decreased in 7 of 13 brain regions. The mean decreases in gray matter were 32% and 15% for rCMRg and rCBF, respectively, resulting in signs of moderate luxury perfusion in some brain areas including the pre- and postcentral gyri, insula, and anterior and posterior cingulate. Though the reduction in CMR is less pronounced than those reported with volatile anesthetics, the metabolic pattern produced with xenon resembles those produced with volatile anesthetics rather than with N 2 O. , ,
During steady state xenon 70% inhalation in rats, having achieved stable cardiovascular conditions, mean values of CBF and CMRg did not change from conscious control values, but during short inhalation of xenon 70%, CBF increased by 40–50%. In pigs, xenon 79% increased rCBF approximately 40% more than total intravenous anesthesia control. However, xenon (30–70%) was reported to have no effect on rCBF and autoregulation in pigs sedated with propofol. It should be noted that MAC of xenon varies among species (71% in humans, 98% in monkeys, 119% in pigs, 85% in rabbits, 161% in rats), and this variation may explain the different results among the species.
The effects of xenon on ICP in patients with head injury are variable; ICP has been found to either increase by 7 mmHg or not to change with xenon 0.45 MAC. In animals either with normal ICP or with elevated ICP, , xenon (0.34–0.7 MAC) did not change ICP. At present, in humans, xenon appears to be a mild cerebral metabolic depressant and its effect on CBF and ICP is mild. In a newborn global hypoxic- ischemic pig model under propofol and remifentanil anesthesia, post-insult administration of xenon reportedly preserves autoregulation.
Because xenon is an antagonist of the NMDA receptor, it may have neuroprotective effects. Indeed, neuroprotection by inhalation of xenon before injury was demonstrated in both in-vitro and in-vivo cerebral ischemia , and traumatic brain injury models, the effect being observed even with post-treatment after hypoxic-ischemic insult in neonatal rats and after traumatic brain injury in adult mice. In combination with either hypothermia (35 °C) or the α 2 – adrenergic agonist dexmedetomidine, xenon exhibited neuroprotection in the same model. Also, it was reported that preconditioning by xenon (70%) reduced brain damage from hypoxia-ischemia in neonatal rats. A later study has demonstrated that xenon (50%) provides long-term neuroprotection in a neonatal hypoxia-ischemia model and that this protection is augmented by concomitant application of moderate hypothermia (32 °C). In a rat transient middle cerebral artery occlusion model, the combination of 30% xenon and subtherapeutic hypothermia (36 °C) after reperfusion has also been demonstrated to provide long-term neuroprotection. However, xenon was also reported to cause neuronal cell death in an in-vitro model of the developing rodent brain at 1 MAC, as does isoflurane and sevoflurane at similarly potent concentrations.
With its low blood/gas partition coefficient of 0.115, xenon may offer advantage for neuroanesthesia use, because early neurologic examination after the emergence period is possible and may be desirable. However, this agent’s effects when combined with other anesthetics should be further determined.
Halothane
Most studies demonstrated that halothane induces cerebral vasodilation and increases CBF, provided that the systemic blood pressure is maintained. The increase in cortical CBF appears greater with halothane than with isoflurane at equi-MAC concentrations. It is probably true that the potency of overall vasodilating property of halothane appears to be most prominent among available volatile anesthetics.
A dose-related cerebral metabolic depressive effect of halothane has been demonstrated repeatedly. At clinical levels of anesthesia, the decrease in global CMRO 2 ranges from 10% to 30%. A study using PET demonstrated that halothane anesthesia titrated to a point just beyond the loss of consciousness is associated with a 40% reduction of whole-brain glucose metabolism, the magnitude of reduction being similar to that with isoflurane.
Halothane raises ICP in a dose-related fashion, and the rise in ICP is parallel to that in CBF. The elevation of ICP with halothane appears to be most prominent among the commonly used volatile anesthetics. However, at 0.5 MAC or less, the effect on ICP is minimal. The increased ICP that, with halothane, often occurs in association with systemic hypotension results in reduced CPP. This response may augment the risk of cerebral ischemia. The increase in ICP may be attenuated either by hyperventilation or by barbiturates. However, the beneficial effects of hypocapnia may not be obtained when the initial ICP is very high or reactivity to CO 2 is lost globally.
In summary, although halothane in low concentrations (less than 1%) can be safely used in clinical neuroanesthesia practice when PaCO 2 is reduced and barbiturates (and probably propofol) also are given, the margin of safety is probably wider with isoflurane, desflurane, or sevoflurane than with halothane.
Isoflurane
In general, the increase in global CBF is smaller with isoflurane than with halothane. However, at a given level of metabolic rate, isoflurane possesses greater cerebral vasodilating capabilities than halothane. It has been demonstrated that halothane, isoflurane, and desflurane at 0.5 MAC produces a similar increase in Vmca in humans during propofol-induced isoelectric EEG, whereas at 1.5 MAC, isoflurane and desflurane have greater vasodilating effects than halothane. The net effect of inhalational anesthetics on CBF is a balance between a reduction in CBF caused by CMR suppression and augmentation of CBF due to direct cerebral vasodilation; the reported smaller increases in CBF with isoflurane than with halothane may occur from a more potent cerebral metabolic depressive effect of isoflurane. The hypothetical illustrations of changes in CBF with rising concentrations of halothane and isoflurane are shown in Fig. 4.2 .
In PET studies in humans, isoflurane (0.2–1.0 MAC) was reported to produce no change in global CBF but to cause regional increase (anterior cingulate and insula regions) and decrease (cerebellum, thalamus, and lingual gyrus) in relative CBF. Additionally, isoflurane (0.5%) was reported to reduce whole brain metabolism by almost 50%, and this reduction was fairly uniform throughout the brain.
It is unlikely that any single mechanism is entirely responsible for the vasodilative property of isoflurane. Some investigators speculate that the vasodilative property of isoflurane may be related to NO. It was also reported that approximately one-third of the cortical hyperemic response to isoflurane measured by laser Doppler flowmetry is mediated by NO, prostaglandins, and epoxyeicosatrienoic acid, and that the remaining part of the response appears to be mediated by a direct action on smooth muscle. A study using a closed cranial window model demonstrated that an adenosine triphosphate (ATP)-sensitive K + channel blocker, glibenclamide, attenuates isoflurane (and sevoflurane)-induced cerebral vasodilation, suggesting that vasodilation with these anesthetics is mediated, at least in part, via activation of ATP-sensitive K + channels.
Gray matter rCBV is increased with isoflurane when the systemic blood pressure is maintained (0.45%), and thus ICP can increase. Data on ICP appear to be inconsistent, and lumbar cerebrospinal fluid pressure (CSFP) increased in one study, while ICP did not change in another. Because ICP was reported to be lower in the patients anesthetized with propofol- fentanyl than those anesthetized with isoflurane-fentanyl, propofol-fentanyl may be preferable in the setting of unstable ICP. In the intensive care unit (ICU) setting, two studies have showed that isoflurane (0.5–0.8 MAC) does not cause a clinically relevant increase in ICP in patients with subarachnoid hemorrhage, intracerebral hemorrhage, or ischemic stroke if baseline ICP values are low or only moderately elevated. ,
Because of the potent cerebral metabolic depressive effect, isoflurane was predicted to have cerebral protective effects, which may be produced by a variety of the mechanisms, including inhibition of excitatory neurotransmission, potentiation of GABA A receptor, regulation of intracellular calcium responses, and activation of TWIK (tandem of P domains in a weak inwardly rectifying K + channel)-related K + (TREK)-1 two-pore-domain K + channels. Indeed, many animal studies demonstrated the neuroprotective properties of isoflurane within clinically relevant concentrations. However, the protection with isoflurane is only applicable to mild insults, being inferior to and less durable than mild hypothermia in its neuroprotective effect. Also, it is important to note that isoflurane has preconditioning , and postconditioning effects. In the developing brain, the neurotoxicity of isoflurane has been reported.
In the clinical setting, there is no good evidence that isoflurane uniquely protects against ischemic central nervous system injury. Suggestive observations include those in a large retrospective review of changing anesthetic management practices during carotid endarterectomy in humans. The critical CBF below which ischemic EEG changes occur was greater in patients anesthetized with halothane than in patients anesthetized with isoflurane. At a comparable level of rCBF, the incidence of EEG ischemic changes with isoflurane has been reported to be significantly lower than that seen with halothane.
In summary, isoflurane appears to produce a mild increase in CBF and a pronounced decrease in cerebral metabolism. The accumulated evidence in basic research strongly suggests that isoflurane has a cerebral protective effect, although it is not proven clinically. However, isoflurane may be a desirable anesthetic for many neurosurgical procedures, including carotid endarterectomy. The increase in ICP caused by isoflurane, if it occurs, may be mild and can be prevented by hypocapnia. However, when ICP elevation should definitely be avoided, propofol in combination with synthetic opioid may be preferable.
Sevoflurane
Studies using PET demonstrated that either a decrease or no change in global CBF occurred with the use of sevoflurane. MAP decreased significantly with sevoflurane in the former study, whereas MAP was unchanged in the latter study. Therefore, it seems likely that global CBF is unchanged with sevoflurane when MAP is maintained. Although global CBF did not change, heterogeneity of the rCBF response was observed; an increase of relative rCBF in the anterior cingulate and a decrease in the cerebellum. Regional heterogeneity of the cerebrovascular response to hyperventilation was also observed with the use of sevoflurane 1 MAC, with the greatest values being observed in the thalamus.
The results obtained with the use of the transcranial Doppler technique revealed that sevoflurane at both 0.5 and 1.5 MAC produced smaller increases in Vmca than isoflurane during propofol-induced isoelectric EEG, suggesting that sevoflurane has less vasodilating effects than isoflurane. Vmca varies according to age. In adults, Vmca was reported to decrease with the use of sevoflurane (0.5–1.5 MAC) despite no change of MAP. , A study of the use of sevoflurane 3% in infants younger than 2 years showed that Vmca was unchanged until MAP dropped below 60% of baseline in infants older than 6 months. However, in infants younger than 6 months, Vmca started to decrease when MAP dropped below 80% of baseline.
Sevoflurane 1 MAC was reported to decrease rCMRg in all regions of the human brain, with the most marked suppression in the lingual gyrus (by 71%), occipital lobe in general (by 68%), and thalamus (by 68%). CBF equivalent (an index of flow–metabolism relationship) was shown slightly higher than normal but was comparable to or slightly lower than the values of equi-MAC isoflurane. Taken together, studies show that sevoflurane decreases CMR but its effect on CBF seems weaker than that of isoflurane.
Sevoflurane, either with or without N 2 O, produced no or small increases in ICP in animals and humans. These effects seem to be consistent with the fact that sevoflurane does not affect CBV even though it reduces CBF. The increase in ICP, if any, can be blocked by hyperventilation. When sevoflurane is compared with isoflurane and desflurane, the extent of the increase in ICP is in the following order in animals: desflurane > isoflurane > sevoflurane. In patients undergoing transsphenoidal pituitary surgery (no mass effect), lumbar CSFP was increased with sevoflurane, but mean increase was small (by 2 mmHg) and comparable to those reported with isoflurane and desflurane. In patients with cerebral tumors (midline shift less than 10 mm) anesthetized with propofol-fentanyl, isoflurane-fentanyl, or sevoflurane-fentanyl, the values of ICP were in the order of propofol-fentanyl < isoflurane-fentanyl = sevoflurane-fentanyl anesthesia. The effects of propofol-fentanyl were less than those of isoflurane-fentanyl and sevoflurane-fentanyl anesthesia. The ICP raising properties of a clinical dose of sevoflurane appear to be mild, but propofol would be preferable in a patient for whom ICP must be rigidly controlled.
The neuroprotective effect of sevoflurane reported earlier in middle cerebral artery occlusion (MCAO) model in rats may be assumed to be due to temperature reduction with anesthetics. However, several other studies demonstrated neuroprotective effects, and the agent’s favorable effect appears to be similar to that of isoflurane. In addition, sevoflurane- induced early or late ischemic tolerance has been shown in rats. However, neurotoxic effects of sevoflurane, as in isoflurane, in the developing brain have been reported.
In the clinical setting, there is no unequivocal evidence of neuroprotective effect of sevoflurane. Indirect evidence includes the observation that critical rCBF, below which ischemic EEG changes occur during carotid cross-clamp, was found to be similar to that previously determined in patients anesthetized with isoflurane. At present, the neuroprotective effects of sevoflurane, though inconclusive, appear to be similar, if they occur, to those of isoflurane.
Desflurane
When using a modified Kety-Schmidt technique with argon, desflurane 1 MAC decreases CBF by 22% in association with decreases by half in CMRO 2 and CMRg by 35% with cerebrovascular CO 2 reactivity being preserved. During propofol-induced isoelectric EEG, desflurane at 0.5 MAC produced increases in Vmca that were similar to those seen with isoflurane. At 1.5 MAC, the increase produced by desflurane was also similar to that with isoflurane. This finding may suggest that desflurane, used when cerebral metabolism is maximally suppressed, increases CBF in a dose-dependent manner. During hypocapnia (PaCO 2 25 mmHg) in patients receiving desflurane, the CBF at 1 MAC was lower than, and the CBF at 1.5 MAC was similar to, those measured during an equi-MAC isoflurane anesthesia. After the induction of anesthesia with propofol, patients receiving desflurane 1.5 MAC had significantly greater Vmca, heart rate, and MAP than those receiving equi-MAC sevoflurane. As desflurane 1.5 MAC was reported to impair autoregulation, it seems likely that the increase in Vmca was caused by the increase in MAP with desflurane.
In patients with supratentorial mass lesions with a midline shift, desflurane 1 MAC (7%) was reported to produce an increase in CSFP despite prior establishment of hypocapnia, but not to raise ICP in patients without a midline shift, whereas isoflurane 1 MAC was reported not to change CSFP or ICP in either case. , In animals subjected to intracranial hypertension, desflurane increased ICP more than sevoflurane but less than isoflurane, whereas during hypocapnia, no significant differences in ICP were observed among three agents.
Many studies have demonstrated neuroprotective effects of desflurane in in-vivo and in-vitro models in experimental animals. The degree of protection afforded by desflurane appears to be comparable to that of isoflurane. , However, the neurotoxic effects of desflurane were also reported in the developing brain of animals. In the clinical setting, brain tissue oxygen pressure measured in patients undergoing craniotomy was elevated when desflurane concentration was increased from 3% to 9%, and the blood pressure was maintained with intravenous phenylephrine.
In summary, because of a low blood/gas partition coefficient (0.42) relative to other clinically used volatile inhalational anesthetics, desflurane can provide rapid onset and offset of anesthesia, which facilitate early neurologic evaluation. In general, desflurane decreases CMR, but CBF may either be increased or decreased, depending on the doses used. Desflurane’s neuroprotective effects appear to be comparable to those of isoflurane. However, because desflurane may have slightly greater ICP-elevating effects than isoflurane or sevoflurane, desflurane should be used cautiously in patients with unstable ICP.
Intravenous Anesthetics
In general, intravenous anesthetics cause a decrease in CBF and CMRO 2 . However, these anesthetics might not be vasoconstrictors in a strict sense, because in-vitro barbiturates, for example, dilate isolated cerebral vessels. The decrease in CBF induced by most intravenous anesthetics appears to be the result of reduced cerebral metabolism secondary to cerebral functional depression. Among the intravenous anesthetics, ketamine may be unique because it produces an increase in both CBF and CMRO 2 . Table 4.2 summarizes the effects of intravenous anesthetics on CBF, CMR, and ICP.
Cerebral Blood Flow | Cerebral Metabolic Rate | Intracranial Pressure | |
---|---|---|---|
Barbiturates | ↓↓ | ↓↓ | ↓↓ |
Etomidate | ↓↓ | ↓↓ | ↓↓ |
Propofol | ↓↓ | ↓↓ | ↓↓ |
Ketamine | ↑↑ | ↑ or → | ↑ or ↑↑ |
Benzodiazepines | ↓ | ↓ | ↓ or → |
Synthetic opioids | → or ➚ ➘ | → or ↓ | → or ➚ |
Dexmedetomidine | ↓ | → or ↓ | → |
Barbiturates
Thiopental produces a dose-dependent reduction in CBF and CMRO 2 . Other barbiturates, such as phenobarbital and pentobarbital, essentially have similar effects. A dose-dependent reduction in CBF and CMRO 2 occurs until the EEG becomes flat. At the point of EEG isoelectricity, no further CMRO 2 reduction occurs despite a further increase in barbiturate dose. A burst suppression dose of thiopental decreases both CBF and CMRO 2 to about 40% (near maximal reduction) of the awake value in humans. Thus, with barbiturates, functional depression appears to be coupled with the reduction in CBF and CMRO 2 . If CMR is maximally depressed as a result of an intracranial pathologic condition, thiopental can increase CBF, possibly because of its direct effect, provided that blood pressure is maintained.
ICP is reduced by barbiturates, possibly through the reduction in CBF and CBV. Barbiturates have been shown to result in lower CBV values than those resulting from use of volatile anesthetics. This effect might be exploited during the treatment of raised ICP in head-injured patients when blood pressure is maintained as well as in the induction of anesthesia in patients with decreased intracranial compliance. Barbiturates attenuate the cerebral vasodilation produced by N 2 O and ketamine.
The neuroprotective effect of barbiturates has been repeatedly demonstrated in focal ischemia models. In a rat model of focal cerebral ischemia (90 minutes), significant reductions in infarct volume have been demonstrated with moderate doses of pentobarbital. Increasing doses sufficient to produce burst suppression on EEG did not further decrease infarct volume. Moreover, in a rat model of focal cerebral ischemia (180 minutes), the burst suppression dose of thiopental, but not methohexital or pentobarbital, reduced infarct volume. It has been suggested that burst suppression of EEG is not necessary to provide neuroprotection and that mechanisms other than metabolic depression may be involved for protection. In humans, post hoc analysis of data acquired as part of the IHAST was not able to show the protective effects of thiopental in patients undergoing temporary clipping during cerebral aneurysm surgery. In the developing brain, the neurotoxicity of pentobarbital was also reported.
ICP-reducing effects and possible neuroprotective effects make the barbiturates favorable drugs for neurosurgical anesthesia, provided that cardiovascular stability is maintained. It should be noted, however, that prolonged use of barbiturate results in tissue accumulation because of its slow metabolism; this effect can lead to delayed emergence from barbiturate anesthesia. Because of similar cerebral hemodynamic effects and a shorter context-sensitive half-life, other intravenous agents, especially propofol, may be more appropriate for this application.
Etomidate
Etomidate does not have cardiovascular side effects. Almost parallel reductions in CBF and CMRO 2 are induced with etomidate. With clinical doses, CBF and CMRO 2 are decreased by approximately 30% to 50%. Reactivity to CO 2 is preserved during etomidate anesthesia. In animals, etomidate, like barbiturates, decreases CMRO 2 progressively until an isoelectric EEG appears. CBF decreases rapidly with the start of etomidate infusion. A maximal decrease in CBF was achieved before the maximal decrease in CMRO 2 . This finding may suggest that etomidate causes vasoconstriction through a different mechanism (possibly by direct action) from that of the barbiturates. Parallel decreases in ICP and CBF were observed.
Etomidate effectively decreases ICP without diminishing CPP. In severely head-injured patients, etomidate decreased ICP while electrocortical activity was present but was not effective when cortical electrical activity was already maximally suppressed. This finding indicates that the decrease in ICP may be caused by the reduction of CBF (and CBV) that is induced by the functional (metabolic) depressant effects of etomidate.
Although etomidate was reported to have a small neuroprotective effect in a forebrain ischemia model (bilateral carotid artery occlusion with hypotension) in rats, most studies failed to show significant protective effects. In fact, poor outcome or larger infarct volume was reported after incomplete cerebral ischemia and middle cerebral artery occlusion, respectively. The injury-enhancing effect of etomidate in middle cerebral artery occlusion has been attributed to its ability to reduce NO levels in the ischemic brain tissue. In addition, in some patients who underwent cerebral aneurysm surgery, etomidate led to cerebral deoxygenation, which was exaggerated with temporal cerebral artery occlusion.
Adverse effects of this drug include adrenocortical suppression and frequent occurrence of involuntary muscle activity and seizure activity. Etomidate should be used with caution in patients who have a history of seizures.
Propofol
Propofol produces dose-related decreases in global CBF by 50–60%. , In PET studies, variation of rCBF reduction has been demonstrated; large decreases occurred preferentially in the medial thalamus, cuneus and precuneus, and posterior cingulate, orbitofrontal, and right angular gyri, which are implicated in the regulation of arousal, performance of associative functions, and autonomic control. A subsequent study revealed that restoration of consciousness by physostigmine was associated with rCBF increases in the thalamus and precuneus of patients anesthetized with propofol. As with barbiturates, the CBF decrease with propofol is attributable to its metabolic depressant effect. As the CBF decrease with propofol is greater than that with sevoflurane, one may consider that propofol is not suitable for ischemic cerebral disease. However, ipsilateral internal carotid artery pressure during carotid endarterectomy and rCBF in the frontal lobe during revascularization surgery for moyamoya disease were reported to be better maintained with propofol than with sevoflurane, probably because cerebral steal phenomenon can be avoided with propofol.
As with the regional variation in CBF decrease, propofol was reported not only to depress CMR but to do so differently according to region. Overall metabolism in the cortex was depressed (by 58%) more than overall metabolism in the subcortical brain areas (by 48%); in the cortical regions, rCMR was significantly lower in the frontal, parietal, and occipital lobes. Several studies demonstrated that the incidence of a decrease in jugular bulb venous hemoglobin saturation of less than 50% was higher with propofol than with isoflurane- nitrous oxide or sevoflurane, suggesting that cerebral oxygen balance during propofol-based anesthesia can be impaired. However, a study measuring rCBF and rCMRg in the same volunteers demonstrated that the magnitude of the decrease in rCMRg generally exceeded the reduction in rCBF, resulting in signs of moderate luxury perfusion in some brain regions.
In patients with cerebral tumors with midline shift less than 10 mm, ICP was reported to be lower and CPP higher in patients anesthetized with propofol than in those anesthetized with isoflurane or sevoflurane. Nevertheless, in most circumstances, MABP is decreased with propofol. Therefore, if propofol is given to treat intracranial hypertension, like any other drug, attention should be paid to maintaining MABP (CPP). CO 2 reactivity is preserved with propofol. Thus, hyperventilation can decrease ICP during propofol anesthesia.
Regarding the neuroprotective effects of propofol, many studies in animals, , but not all, , proved favorable effects with burst suppression doses of propofol. The possible neuroprotective mechanisms include reduction of the CMR, antioxidant activity, activation of GABA receptors, attenuation of glutamate-mediated excitotoxicity, prevention of mitochondrial swelling, interaction of the endocannabinoid system, attenuation of autophagy activation, downregulation of aquaporin 4 expression, and inhibition of nicotinamide adenine dinucleotide phosphate oxidase. Interestingly, protection was observed even in a light depth of propofol anesthesia (not a burst suppression dose) and even when propofol was administered after ischemic insults. In the developing brain and in traumatic brain injury, the neurotoxicity of propofol was reported. , There is as yet no definitive clinical evidence showing an improvement or impairment of neurological outcome following acute cerebral injury in patients. As propofol has a rapid onset and offset of action with minimal interference with electrophysiological monitoring including motor evoked potentials, propofol seems to be a favorable anesthetic for various types of neurosurgery.
A number of case reports suggest that prolonged use of propofol caused systemic acidosis and progressive cardiac failure and even death in children. , Special attention should be paid when prolonged infusion of propofol is necessary, even in adult patients.
In summary, propofol seems to have cerebral hemodynamic and metabolic effects similar to those of barbiturates. This drug may be useful in the treatment of patients with intracranial pathologic conditions, provided that hypotension is prevented.
Ketamine
Intravenous ketamine (3 mg/kg) increases global CBF by approximately 60%, but global CMRO 2 does not change significantly. PET studies showed that subanesthetic doses of ketamine increased rCBF and rCMRg without a global change in rCMRO 2 ; , the greatest rCBF increases were seen in the anterior cingulate, thalamus, putamen, and frontal cortex, and the greatest rCMRg increases were detected in the thalamus and the frontal and parietal cortices. These data have been obtained with the use of commercially available formulations of ketamine containing both S(+)- and R(-)-ketamine enantiomers. S(+)-ketamine is more effective as an analgesic and anesthetic than a racemic mixture of two enantiomers (racemic ketamine) or R(-)-ketamine. The changes in CBF and CMR with S(+)-ketamine appear essentially similar with minor quantitative difference; subanesthetic doses increased global CBF by 14% without a change in global CMRO 2 , with greatest increase in CBF detected in the anterior cingulate. The anesthetic dose of S(+)-ketamine increased global CBF by 36% without changes in global CMRO 2 or CMRg, with the greatest increase in CBF detected in the insula, whereas CMRO 2 rose only in the frontal cortex, and CMRg increased only in the thalamus. Vasodilation by ketamine has been attributed, in part, to its metabolic stimulating effect, a direct dilating effect, and a cholinergic mechanism. In animals, pretreatment with thiopental completely blocks these CBF and CMRO 2 effects of ketamine, and diazepam pretreatment attenuates the increase in lCMRg in the hippocampus.
Ketamine markedly raises ICP. An increase in ICP can be blocked or attenuated by induced hypocapnia or by administration of thiopental or benzodiazepine. During propofol sedation in patients with traumatic brain injury, ketamine decreases ICP. In patients with supratentorial tumor who are anesthetized with isoflurane (0.3% to 0.4%)/N 2 O 50%, ketamine 1 mg/kg did not significantly raise ICP. However, it was reported that midazolam (0.15 mg/kg) or diazepam (0.2 mg/kg) administered 1 minute before the administration of ketamine (1 mg/kg) was unable to block ketamine-induced ICP elevation at the induction of general anesthesia. Thus ketamine may not be a first choice at the induction of general anesthesia, especially in patients with elevated ICP or decreased intracranial compliance.
Some animal experiments, but not all, demonstrated some neuroprotective effects of ketamine in various intracranial pathologic conditions, including ischemia and head injury, which could be related to NMDA receptor antagonism. Ketamine has also been shown to have potent anti-inflammatory effects. However, improved outcomes in animal experiments were reported only in studies with brief recovery observation intervals. In the developing rodent brain, ketamine was reported to induce neurotoxicity. Clinical studies comparing ketamine sedation with fentanyl or sufentanil after traumatic brain injury failed to find any favorable effects on functional outcome after 6 months. , A definite neuroprotective effect of ketamine remains to be demonstrated in the clinical setting. There is a growing body of evidence that a low dose of ketamine (0.5 mg/kg) rapidly (within hours) improves the core symptoms of depression and the effect is sustained for 7 to 10 days. This effect seems to be associated with increases in the number and function of synaptic connections.
Benzodiazepines
Diazepam in combination with fentanyl and N 2 O produces parallel decreases in CBF and CMRO 2 . In the head-injured patient, diazepam produces proportional 25% decreases in CBF and CMRO 2 . Contrary to the assumption that ICP would be decreased because of a lower CBF, diazepam (0.25 mg/kg) does not change ICP.
Midazolam, like diazepam, produces parallel reductions in CBF and CMRO 2 . With rising doses, the effects appear to reach a plateau, possibly reflecting saturation of the benzodiazepine receptors. The effect of midazolam is completely blocked by the specific benzodiazepine antagonist flumazenil. The PET study demonstrated that midazolam decreased global CBF by 12% and that the decrease in rCBF occurred in the areas associated with the functioning of arousal, attention, and memory, such as the insula, the cingulated gyrus, the prefrontal cortex, the thalamus, and parietal and temporal association areas. Midazolam, even at a very low dose (0.03 mg/kg), appears to decrease rCBF in the left dorsolateral prefrontal cortex, left cingulate gyrus, and left posterior cingulate gyrus/precuneus. Midazolam was reported to preserve CO 2 responsiveness and improve dynamic cerebral autoregulation.
Midazolam produces either a decrease or no change in ICP. Negative results may be due to normal ICP before the administration of the drugs. In patients with severe head injury, there was no significant difference in ICP values between midazolam and propofol. Midazolam has been shown to maintain hemodynamic stability better than thiopental. However, caution must be used because of the possibility of CPP reduction in patients with critical conditions.
Midazolam may have protective effects against hypoxia or cerebral ischemia; the effects appear to be comparable with or slightly less than those of barbiturates in animals. Lorazepam, triazolam, and flurazepam seem to have effects similar to those of diazepam and midazolam. In the developing brain, anesthesia with midazolam, isoflurane, and N 2 O was reported to cause widespread apoptosis.
Because a specific receptor antagonist is now available, benzodiazepine derivatives are useful as induction or supplemental drugs during neuroanesthesia. However, flumazenil, a competitive benzodiazepine receptor antagonist, also antagonizes the effects of midazolam on CBF, CMRO 2 , and ICP. Thus one must use this drug cautiously when reversing benzodiazepine- induced sedation in patients with impaired intracranial compliance. Flumazenil-induced seizure probably produced by unmasking the anticonvulsant effect of benzodiazepine might also be considered.
Synthetic Opioids
The reported effects of synthetic opioids on CBF, CMRO 2 , and ICP are variable. The variability appears to be due to the background anesthetic and opioid dose. When vasodilating drugs are used as the background anesthetic, the effect of the opioid is consistently that of a cerebral vasoconstrictor. Conversely, when a vasoconstrictor is used as the background anesthetic or when no anesthetic is given, opioids either have no effect or even increase CBF. Large doses of opioids decrease CBF in the absence of background anesthetics. , When N 2 O is also used, most opioids decrease CMRO 2 . Variable ICP effects also depend on the background anesthetic and on the systemic blood pressure autoregulation status. Cerebrovascular autoregulation and CO 2 reactivity is preserved with opioids.
Fentanyl and Sufentanil
The combined use of fentanyl (5 μg/kg) and droperidol (0.25 mg/kg) has no significant effect on CBF and CMRO 2 . A large dose of sufentanil (10 μg/kg + 0.15 μg/kg/min) was reported to decrease CBF and CMRO 2 by 29% and 22% in cardiac patients, respectively. Increases in Vmca by approximately 25% were observed when fentanyl (16 μg/kg) or sufentanil (1.7 μg/kg) was used in unpremedicated humans. In premedicated patients, Vmca was not changed with fentanyl (25 μg/kg) or sufentanil (3 μg/kg), but was decreased with sufentanil (6 μg/kg) by 27–30%. This difference suggests that the CBF response depends on background state and the dose of agents. Sufentanil (1.5 μg/kg) decreased Vmca in patients with elevated ICP, and the decrease can be explained by low CPP. A PET study in awake humans showed that fentanyl (1.5 μg/kg) showed heterogeneous changes in rCBF; rCBF increased in the anterior cingulate and contralateral motor cortex and decreased bilaterally in the thalamus and posterior cingulate.
In rats, high doses (200–400 μg/kg) of fentanyl induce seizures, increase CBF in most structures throughout the brain, and activate subcortical brain metabolism. Whether the cerebral metabolism is compromised during seizures to the extent that ischemic brain damage occurs is not yet determined. In humans, the clinical significance of seizure activity observed with fentanyl in rats is not clear.
Earlier studies show that ICP is either not elevated or may be slightly decreased with fentanyl used alone or in combination with droperidol. Reported ICP increases in patients with space-occupying lesions have been attributed to hypercapnia. Herrick and colleagues reported that fentanyl, sufentanil, and alfentanil did not affect brain retractor pressure in hyperventilated neurosurgical patients anesthetized with isoflurane, suggesting that these opioids appear safe for intraoperative administration after the cranium is open. Better cerebral relaxation has been noted when fentanyl or sufentanil was used in patients anesthetized with N 2 O and isoflurane during craniotomy, suggesting that both fentanyl and sufentanil probably have cerebrovasoconstrictive activity.
However, some reports showed that fentanyl and sufentanil increased ICP (or CSFP) in patients with severe head trauma. Werner and colleagues reported that when MABP was controlled and remained unchanged, sufentanil (3 μg/kg) had no significant effects on ICP in patients with brain injury, whereas the same dose of sufentanil caused a transient increase in ICP and a decrease in MABP. From these results, an increase in ICP appears to be attributable to the autoregulatory response, the decrease in cerebrovascular resistance secondary to CPP reduction. However, in one study, there were no differences in the ICP-elevating effect of fentanyl (1.5 μg/kg) between patients with head trauma who had preserved autoregulation and those who had impaired autoregulation. Thus, the cerebrovascular autoregulation may not be the only probable mechanism responsible for fentanyl- induced increases in ICP in patients with head trauma. Although an ICP rise may be only transient after bolus administration of fentanyl and sufentanil, attention should be paid to this effect in patients with unstable ICP.
Alfentanil and Remifentanil
Vmca and ICP did not change with low (25 μg/kg) and high (50 μg/kg) doses of alfentanil in patients anesthetized with isoflurane and N 2 O, provided that MAP was maintained. A low dose of remifentanil (0.1 μg/kg/min) increased rCBF in the white and gray matter in awake humans. In contrast, large doses of remifentanil (2 and 4 μg/kg/min) decreased rCBF in humans who received 0.5–1 mg/kg propofol to facilitate laryngeal mask insertion. In cardiac patients without background anesthetics, moderate doses of remifentanil (2 μg/kg IV + 1 μg/kg/min) did not change Vmca, whereas large doses (5 μg/kg IV + 3 μg/kg/min) decreased Vmca by 31%, despite no change of MAP.
Both alfentanil and remifentanil essentially have a minimal effect on ICP. As mentioned earlier, alfentanil did not affect brain retractor pressure in hyperventilated neurosurgical patients anesthetized with isoflurane. No ICP elevation with alfentanil was observed in pediatric patients with hydrocephalus who were anesthetized with isoflurane and N 2 O. However, in an experimental brain injury model, Souter and colleagues reported that rapid infusion of alfentanil increased ICP concomitant with reduction in MABP but with no CBF changes.
In a PET study in human volunteers, either increases or decreases in relative rCBF were observed with an infusion of low doses of remifentanil (0.05 μg/kg/min), depending on the structures, the increase being observed within structures involved in pain processing. With moderate doses (0.15 μg/kg/min), changes in relative rCBF were observed in structures involved in modulating vigilance and alertness. The increases in relative rCBF with painful heat stimulation detected in various structures, including the thalamus, were suppressed with rising remifentanil dosage (0.05 to 0.15 μg/kg/min), whereas relative rCBF rose in the cingulofrontal cortex and periaqueductal gray, where descending antinociceptive pathways exist.
In summary, clinically used doses of most opioids have minimal to modest depressive effects on CBF and CMRO 2 . During opioid-induced seizures in animals, there are a substantial increase in CBF and activation of subcortical brain metabolism, although they were not seen in humans. If adequate alveolar ventilation is instituted to maintain PaCO 2 (and PaO 2 ) within the normal range and rigidity is prevented, clinical doses of opioids have minimal or negligible effects on ICP. However, the possibility of an increase in ICP with synthetic opioids cannot be completely excluded. Whenever these opioids are used, slow administration and care to maintain MABP are recommended. It seems probable that remifentanil with either propofol or dexmedetomidine may be a useful regimens for various neurosurgical procedures, including minimally invasive surgery.
Muscle Relaxants
Succinylcholine
Many studies have demonstrated that succinylcholine elevates ICP in animals and humans irrespective of the presence or absence of space-occupying intracranial lesions. The rise in ICP with succinylcholine was accompanied by muscle fasciculation, an increase in muscle spindle afferent activity, EEG arousal, and an elevation of CBF. Fasciculation in the muscles of the neck, causing stasis in the jugular veins, might also be a factor contributing to increased ICP with succinylcholine. The rise in ICP was prevented or diminished by pretreatment with a nondepolarizing muscle relaxant, the results contrasting with the animal study in which pretreatment with pancuronium did not attenuate the succinylcholine-induced increase in afferent muscle activity and CBF.
Succinylcholine-induced increases in serum K + in a patient with subarachnoid hemorrhage is another concern. It seems independent of the presence of motor dysfunction and may not be significant at a relatively early stage (within 10 days). Nevertheless, the use of succinylcholine has been diminishing in clinical neuroanesthesia practice, with the exception of emergency situations, such as the patient with a full stomach in whom a rapid-sequence induction is recommended. In this situation, prior administration of small doses of nondepolarizing muscle relaxant or lidocaine is recommended.
Nondepolarizing Muscle Relaxants
Some nondepolarizing muscle relaxants or their metabolites may affect the cerebral circulation through a histamine- releasing property that has pharmacologic activity. Clinical dose of atracurium appears to have no significant effect on CBF, CMRO 2 , or ICP. However, high doses of atracurium have the potential to release histamine, though the potential is considerably less than that of d-tubocurarine. Histamine can reduce CPP because of the increase in ICP caused by cerebral vasodilation and the decrease in MAP. The metabolite of atracurium, laudanosine, has been reported to cross the blood–brain barrier readily and cause seizures. However, the blood level of laudanosine after clinical doses of atracurium should not have undesirable consequences. No significant differences in seizure threshold for lidocaine have been reported in cats paralyzed with atracurium, pancuronium, and vecuronium.
Cisatracurium, an intermediate-acting muscle relaxant, produces and releases less laudanosine and histamine than atracurium. The cerebral effects of cisatracurium are essentially similar to or weaker than those of atracurium.
Pancuronium, vecuronium, rocuronium, and pipecuronium have little or minimal effect on CBF, CMRO 2 , or ICP. Pancuronium raises blood pressure and heart rate, which could be disadvantageous for certain patients, such as those with hypertension, especially if they have disturbed autoregulation. In these patients, a substantial elevation of ICP could occur. Vecuronium neither induces histamine release nor does it change blood pressure or heart rate, and thus it may be preferable. Rocuronium, because of its rapid onset of action in comparison with other nondeporalizing muscle relaxants and its lack of adverse activity, such as histamine release, may be preferable to succinylcholine during rapid induction of anesthesia.
In summary, if succinylcholine is used, prior administration of small doses of a nondepolarizing muscle relaxant or lidocaine is recommended, as well as maintenance of adequate depth of anesthesia. With respect to the use of nondepolarizing muscle relaxants, in most clinical situations the changes in CBF and ICP are minimal if respiration is well controlled and an increase in PaCO 2 is avoided. One should be aware of residual neuromuscular blockade after emergence from anesthesia, especially when renal function is compromised, as it could lead to hypercapnia and a concomitant increase in ICP.
Other Drugs
Lidocaine
Lidocaine has unique central nervous system effects that depend on the blood concentration; at low concentration, sedation occurs, but at higher concentration, seizures may occur. Non-seizure-inducing doses of lidocaine produce a dose- related reduction of CMRO 2 and CBF. Large doses of lidocaine reduce CMRO 2 by a maximum of 30% in dogs. If seizures are induced by lidocaine, CMRO 2 increases, as does CBF. Brain oxygenation seems to be adequate. However, regional flow- metabolism imbalance may not be excluded entirely.
Intravenous lidocaine 1.5 mg/kg has been reported to be effective in preventing circulatory changes and an elevation of ICP during tracheal intubation, endotracheal suctioning, or after application of a pin-type skull clamp or skin incision in patients undergoing craniotomy.
Several sodium channel blockers have been investigated and suggested as possible neuroprotectants. Although the protective effect of lidocaine was not demonstrated in severe forebrain ischemia, it was demonstrated in transient focal cerebral ischemia. The dosage regimen was clinically relevant. The mechanism for protection appears to be related to preservation of mitochondrial function, inhibition of glutamate release, and inhibition of apoptosis. A small clinical trial demonstrated a benefit of a clinically relevant dose of lidocaine infusion during cardiac surgery in long-term (6 months) neuropsychological conditions. However, subsequent large-scale, randomized, double-blind studies failed to demonstrate neurocognitive improvement through use of lidocaine. ,
Alpha 2 -Adrenergic Agonists
Dexmedetomidine (0.2–0.6 μg/kg/h) was reported to decrease global CBF by one-third, with rCBF in most of the cortical and subcortical brain regions being decreased. Dexmedetomidine also decreased Vmca in a dose-dependent manner, with the maximum reduction being approximately 25% at the hypnotic doses. Although animal studies demonstrated that dexmedetomidine reduced CBF without a concomitant reduction in CMR, a reduction of the CBF/CMR ratio was not observed in humans. Indeed, dexmedetomidine reduced CMR equivalent (CMRe; this value is calculated by multiplying Vmca by the difference between arterial and cerebral jugular venous oxygen contents) in healthy volunteers in a dose-dependent manner. The decreases in the CBF/CMR ratio that were anticipated from animal studies were not observed. In addition, no clinically significant reduction of brain tissue oxygenation occurred with dexmedetomidine (1 μg/kg + 0.5–0.7 μg/kg/h) in neurovascular surgery patients.
A PET study in humans demonstrated a significant negative linear correlation between clonidine concentration and rCBF in the thalamus, prefrontal, orbital and parietal association cortex, posterior cingulated cortex, and precuneus, suggesting that the pattern of regional deactivation during the sedation induced by clonidine is very close to the pattern of physiologic early stage of non-rapid eye movement (REM) sleep. It was reported in humans that dexmedetomidine was seen to weaken dynamic cerebrovascular autoregulation. In healthy human volunteers, oral administration of clonidine 5 μg/kg decreased Vmca by approximately 20% with slight attenuation of CO 2 reactivity. CO 2 reactivity with dexmedetomidine in humans is preserved or may be impaired. ,
As to the mechanisms of the cerebrovascular effects of α 2 – antagonists, there are several animal studies. Clonidine, topically applied through a cranial window, constricted pial arteries and veins, and this effect was blocked by pretreatment of yohimbine, an α 2 -antagonist. The pretreatment with glibenclamide, a blocker of ATP-sensitive potassium channel, potentiated the vasoconstriction of pial arteries, indicating that α 2 -agonist induced activation of ATP-sensitive potassium channels as a counterbalancing vasodilatory effect. Neither inhibition of NO synthase nor blockade of β-adrenoreceptors affects the cerebral vasoconstriction induced by dexmedetomidine.
In patients with normal ICP after transsphenoidal pituitary tumor surgery, dexmedetomidine (total dose approximately 1 μg/kg) had no effect on lumbar CSF pressure, but decreased MABP and CPP. Also, in severely head-injured patients, a single dose of clonidine (2.5 μg/kg IV) did not significantly affect ICP but significantly reduced MABP and CPP. Some patients displayed a transient increase (> 10 mmHg) in ICP concomitant with a decrease in MABP, which may have resulted from cerebral autoregulatory vasodilation mechanism.
A variety of in-vivo and in-vitro models demonstrated the neuroprotective effects of α 2 agonists, especially dexmedetomidine. Of note, dexmedetomidine was reported to attenuate isoflurane- or ketamine-induced neurotoxicity in the rodent developing brain. , No clinical evidence of neuroprotective effects of dexmedetomidine is available as yet. Nevertheless, because of rapid onset and offset of effective sedation without respiratory depression, dexmedetomidine may be advantageous for awake craniotomy.
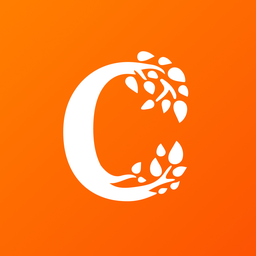
Full access? Get Clinical Tree
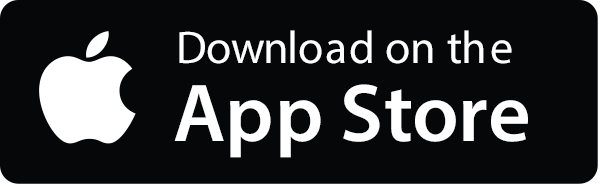
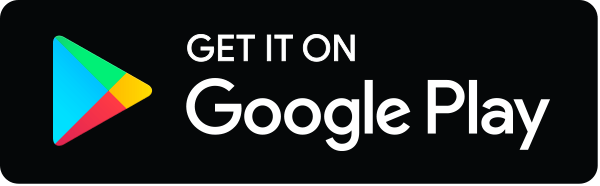