Chapter 77 Diving Medicine
For online-only figures, please go to www.expertconsult.com
There are many millions of recreational, commercial, and military divers worldwide; hundreds of thousands of new divers are trained each year. According to the 2000 U.S. Census Data, approximately 2.5 million active certified divers reside in the United States alone (http://www.allcountries.org/uscensus). Diving is conducted in every imaginable aquatic setting, including oceans, lakes, reservoirs, rivers, quarries, ponds, and aquariums. Despite the extent of diving activity, diving-related fatalities and serious injuries are rare. For recreational divers, the average death rate (based on data derived from case claims of insured divers) is 16.4 deaths (range 12.1 to 22.9) per 100,000 divers per year.57 The chance of suffering decompression sickness (DCS) on any single dive is about 4 in 10,000 in warm water and 59 per 10,000 in cold water.182
The best, and most readily available information about diving accidents comes from the Divers Alert Network (DAN), a private, nonprofit dive safety organization supported by clinical and academic affiliations, including the University of California at San Diego, the University of Pennsylvania, and Duke University. Established in 1980, DAN provides diving medical assistance to the diving community all over the world. Services include dissemination of medical information, evacuation support, and accident insurance. DAN is a member-supported organization with over 200,000 U.S. members and approximately 265,000 members worldwide (http://www.DiversAlertNetwork.org). DAN’s medical hotline number is 919-684-9111.
One of DAN’s key roles in the diving industry is management of dive fatality and accident data. Diving-associated fatalities peaked in the mid 1970s with annual rates as high as 150 and have been stable since that time, with an average of 84 (range 77 to 91) fatalities per year.57 In a recent effort to further define the scope of diving fatalities and overcome the perennial problem of uncertain total numbers (denominators), DAN researchers used claims data from 2000 to 2006 from insured members. In this way, the published incidence rates were based on a known denominator (number of insured). Over that 7-year period, there were 187 death claims among 1,141,367 insured member years, for which the mean annual fatality rate (AFR) was 16.4 (range 12.1 to 22.9) deaths per 100,000 persons.57 Fatality data from the British Sub-Aqua Club are similar for the same period, with 14.4 deaths per 100,000 divers.
DAN hosted a workshop on diving fatalities in Durham, North Carolina, in April of 2010 to address industry concerns about dive accident causation and to solicit input from industry leaders about possible intervention strategies. The proceedings of this meeting are freely available online at www.diversalertnetwork.org. Of interest to the attendees were not just fatalities but their underlying cause. Based on a paper presented by Denoble and colleagues, “The most common disabling injuries associated with death were asphyxia, arterial gas embolism (AGE), and acute cardiac related events. The most common root causes were gas supply problems, emergency ascent, cardiac health issues, entrapment/entanglement, and buoyancy trouble. The risk for death while diving increased with age, starting in the early thirties. This is likely due to the naturally increased prevalence of cardiac disease with age, but an increased association of AGE and asphyxia were also associated with aging.”56
This chapter focuses primarily on the pressure-related diving syndromes collectively known as dysbarism; additional conditions relevant to diving are discussed in other chapters (e.g., immersion hypothermia in Chapter 6, submersion incidents in Chapter 75, hyperbaric oxygen therapy in Chapter 78, and hazardous marine life in Chapters 79 to 81).
Historical Perspective
In the 17th century, primitive bells containing air were carried from the surface, allowing Swedish divers to stay underwater longer than a single breath and to salvage cannons from Stockholm’s harbor.154 In 1690, Sir Edmund Halley devised a leather tube to carry surface air to barrels, which resupplied air to manned bells at a depth of 18 m sea water (msw) (60 feet sea water [fsw]). These barrels were submerged, and the air they contained was compressed.53
The first practical diving suit was fabricated by Augustus Siebe in 1837.3,53,154 Atmospheric air was supplied to the diver as compressed air from a manually powered pump on the surface. By 1841, French engineers had developed the technique of using compressed air to keep water and mud out of caissons sunk to the bottom of riverbeds for bridge footings and tunnels. Soon thereafter, it was noted that people working in a compressed-air environment sometimes suffered joint pains, paralysis, and other medical problems soon after leaving the caisson. This poorly understood condition was called “caisson’s disease” and was the first recognition of what is now known as decompression sickness.158
In 1865, the French engineers Rouquayrol and Denayrouse developed a device that could supply air on demand at ambient pressures different from the 1 atmosphere of pressure found at sea level. These inventors were able to supply air on demand at appropriate breathing pressure to persons underwater with a “demand regulator,” as it subsequently became known. This device originally required a surface air supply connection.3 The demand valve regulator was later modified to supply auxiliary oxygen for pilots operating at high altitude. In 1943, while working with the French resistance against Nazi Germany, Jacques-Yves Cousteau and Emile Gagnon combined a demand valve regulator with a compressed air tank, giving rise to what they called “self-contained underwater breathing apparatus,” or scuba.
The potential military usefulness of scuba was immediately recognized and led to a considerable amount of investigation during World War II. As initially configured, scuba was used in an open-circuit mode in which exhaled air was simply vented into the water. This was wasteful of the compressed air supply and had other disadvantages for military uses. Further work led to refinement of rebreather scuba devices (both closed- and semiclosed-circuit systems), such as Lambertsen’s amphibious respiratory unit.11 These rebreather systems conserved the breathing gas by using a carbon dioxide scrubber and recirculating all or part of each exhalation (see Rebreather Diving, later). These specialized scuba systems were useful for military purposes because they allowed longer submergence times and could be used in clandestine operations or when disarming pressure-sensitive explosive devices. However, they had a greater frequency of mishap, so rebreather systems were not widely used until the 1990s, when there began a resurgence of interest in them by “technical” recreational divers.
After World War II, development and marketing of open-circuit scuba equipment to the general public made the underwater world accessible to growing numbers of people. In the last four decades, scuba diving has opened the underwater world to millions of divers and hundreds of millions of cinema observers. Scuba is now used as a basic tool with myriad commercial, military, scientific, and recreational applications (Box 77-1).7
Types of Diving and Diving Equipment
Breath-Hold Diving
Today, competitive freediving attracts athletes from all over the world and is regularly featured on sports television channels. The sport is governed by the Association Internationale pour le Developpement de l’Apnée—also known as the International Association for the Development of Apnea (AIDA; http://www.aida-international.org/) or the International Association for the Development of Freediving. AIDA has been the officiating body for freediving, setting standards and recognizing records since 1992. AIDA recognizes eight types of freediving and breath holding; descriptions of these disciplines and the world records for reach can be found in Table 77-1.
Medical Problems of Breath-Hold Diving
The major medical concern of breath-hold diving is development of hypoxia leading to loss of consciousness and drowning, especially if submergence is preceded by hyperventilation (see Hyperventilation and Shallow Water Blackout, later). Breath-hold divers also may become hypothermic, get entangled in underwater debris (e.g., fishing line, ropes, and cables) or vegetation, be harmed by marine animals, or be injured by boats or other watercraft. Divers are also subject to barotrauma of the ears and sinuses, as described later. Although it is very rare, breath-hold divers also can suffer from decompression sickness (see Decompression Sickness, later).
Rebreather Diving
All rebreathers have certain elements in common, and they fall into one of four general categories of devices:8
Mixed-Gas Diving
Enriched Air Nitrox
Overall, use of nitrox for recreational diving purposes is still evolving. Clearly, it has advantages and disadvantages (Box 77-2), with the advantages most likely realized in a setting that ensures adherence to safety.
Heliox
Because it causes negligible, if any, narcosis and is easier to breathe at greater depths (because of lesser density), heliox is the preferred gas used in commercial diving at depths over 130 fsw. The major problems with helium are its expense (which precludes its widespread use in recreational diving), greater thermal conductivity, and hindrance of speech. In commercial and military settings, helium speech unscramblers are typically used. However, at depths over 183 msw (600 fsw), and especially with rapid descent, helium causes a poorly understood condition known as high-pressure nervous syndrome (HPNS). First noted in the 1960s, HPNS is characterized by dizziness, nausea, vomiting, postural and intention tremors, fatigue, somnolence, myoclonic jerking, stomach cramps, numbness, and sleep disturbances.16 HPNS is a major barrier to manned undersea activity deeper than 183 msw (600 fsw).
Saturation Diving
The need to minimize prolonged decompression after deep diving led to development of saturation diving. In the late 1950s, experiments by USN diving medical officers George Bond and Robert Workman coincided with those of Jacques-Yves Cousteau and Edward Link in the commercial sector, all of whom were working on ways to stay underwater at great depths long enough to perform useful work.3
One-Atmosphere Diving
One-atmosphere absolute (ATA) diving systems are, in essence, small submarines with various types of propulsion systems and manipulators that allow the operator to work at great depth. The interior of the unit is maintained with environmental control systems to retain safe physiologic parameters. These systems range from one-person ATA suits in which a diver can “walk” (Figure 77-1, online) to submersibles that accommodate two or more occupants.
Diving Physics
Divers encounter many adverse environmental conditions underwater. These include cold, changes in light transmission and sound conduction, lack of air to breathe, increased density of the surrounding environment, and increased atmospheric pressure. Not surprisingly, diverse medical problems are related to diving (Box 77-3).
BOX 77-3 Medical Problems of Scuba Divers
Pressure change with increasing depth is linear, although the greatest relative change in pressure per unit of depth change occurs nearest the surface. Table 77-2 lists commonly used units of pressure measurement in seawater.
When a diver submerges, the force of the tremendous weight of the water above is exerted over the entire body. Except for air-containing spaces such as the lungs, paranasal sinuses, intestines, and middle ears, the body behaves as a liquid. The law that describes the behavior of pressure in liquids is named for the 17th-century scientist, Blaise Pascal. Pascal’s law states that a pressure applied to any part of a fluid is transmitted equally throughout the fluid. Thus, when a diver reaches 10 msw (33 fsw), the pressure on the surface of the skin and throughout the body tissues is 29.4 psia or 1520 mm Hg (Figure 77-2). The diver’s body is generally unaware of this pressure, except in the air-containing spaces of the body. The gases in these spaces obey Boyle’s law (Figure 77-3), which states that the pressure of a given quantity of gas for which temperature remains unchanged varies inversely with its volume. Thus, air in the middle ear, paranasal sinuses, lungs, and gastrointestinal tract is reduced in volume during compression or descent underwater. Inability to maintain gas pressure in these body spaces equal to the surrounding water pressure leads to various untoward mechanical effects, which are discussed later.

FIGURE 77-2 Pascal’s law. Pressure applied to any part of a fluid is transmitted equally throughout the fluid.
Barotrauma
Barotrauma of Descent
Mask Barotrauma
For humans to see underwater, an air space must be present between the eyes and water. In scuba diving, this is created using a face mask consisting of tempered safety glass in a soft malleable mask that seals across the forehead, on the sides of the face, and under the nose to allow nasal exhalations into the mask space to maintain air pressure inside the mask. As a diver descends in the water, the ambient pressure increases and air must be added to the gas inside the face mask to equalize water pressure. If inexperience or inattention cause the diver to forget to maintain this balance, negative pressure in the mask can rupture capillaries, causing skin ecchymosis, subconjunctival hemorrhage, lid edema, and rarely hyphema. This unusual condition is known as mask squeeze (Figure 77-4; see also Figure 28-20).

FIGURE 77-4 Mask barotrauma. A novice diver with mask barotrauma showing subconjunctival hemorrhages.
Orbital hemorrhage from face mask barotrauma is an unusual complication and can be associated with diplopia, proptosis, and visual loss.35,123,163 Although such neurologic findings after scuba diving may suggest arterial gas embolism or neurologic decompression sickness, the presence of the unmistakable stigmata of mask squeeze and a consistent history should prompt consideration of orbital hemorrhage. Under these circumstances, instead of immediate referral to a recompression facility, the diver should be referred for immediate magnetic resonance imaging (MRI) and ophthalmology consultation, because of the possibility of permanent vision loss caused by compression of the optic nerve or elevated intraocular pressure. In rare cases, surgery intervention may be necessary.163 Recompression is contraindicated for orbital hemorrhage unless the diver also suffers from arterial gas embolism or decompression sickness.
Sinus Barotrauma
The four paired paranasal sinuses—frontal, maxillary, ethmoid, and sphenoid—have narrow connections to the nasal cavity via the sinus ostia. If there is inability to maintain the air pressure in any paranasal sinus during descent, a relative vacuum develops in the sinus cavity. This negative pressure causes congestion of the mucosal lining with subsequent edema and intramucosal bleeding and possible hematoma, hemorrhagic bullae, and bleeding into the sinus (Figure 77-5). In cases of sinus squeeze, the diver usually experiences increasingly severe pain over the affected sinus during descent. On ascent, the remaining gas in the sinus expands and may force mucus and blood into the nose and mask.

FIGURE 77-5 Frontal sinus barotrauma that first occurred 3 days earlier. Note the persistent air-fluid level.
(Courtesy Kenneth W. Kizer, MD.)
The frontal sinus is most commonly affected by barotrauma, followed by the maxillary sinus. With maxillary sinus involvement, the diver often experiences pain in the maxillary teeth caused by compression of the posterior superior branch of the fifth cranial nerve, which runs along the base of the maxillary sinus. Additionally, maxillary sinus barotrauma can cause compression or ischemic neuropraxia of the infraorbital branch of the fifth cranial nerve, causing tingling and numbness of the cheek and upper lip.34,148 Other complications include sinusitis from infection of the intrasinus fluid, or periorbital emphysema from air dissecting through the lamina papyracea from the ethmoid sinus into the orbits.
Sinus barotrauma usually occurs in the setting of a diver who has an upper respiratory infection or severe allergies or who has an anatomic deformity such as nasal polyps or a deviated septum. Divers with a history of sinusitis or middle-ear barotrauma may be more prone to paranasal sinus barotrauma.180 Consequently, prevention of sinus barotrauma includes avoidance of diving when suffering from an upper respiratory infection, while symptomatic from allergic rhinitis, and when sinusitis, nasal polyps, or any other condition is present that impairs free flow of air from sinus cavity to nose. Significant nasal deformity may predispose to sinus barotrauma and may warrant surgical correction to allow one to continue diving.
Middle Ear Barotrauma (Barotitis Media)
Referred to in diver parlance as ear squeeze, barotitis media is the most common medical problem in scuba diving, probably affecting more than 40% of divers at one time or another.91 The problem can be explained by a direct application of Boyle’s law (Figure 77-6), potentially compounded by the structure of the eustachian tube.

FIGURE 77-6 Middle ear barotrauma. Symptoms include fullness and pain caused by stretching of the tympanic membrane.
If a diver does not heed the initial symptoms of barotitis media and allows the pressure differential to reach 100 to 400 mm Hg (i.e., at depths of 1.3 to 5.3 msw [4.3 to 17.4 fsw]), the pressure imbalance may lead to rupture of the tympanic membrane.82 In such a case, the problem for the diver may be compounded by entry of cold water into the middle ear. This may cause severe vertigo.
Otoscopic appearance of the tympanic membrane in cases of barotitis media varies with the severity of the injury. A commonly used grading scheme is the Teed classification, which grades severity according to the amount of hemorrhage in the tympanic membrane (Table 77-3).74 Each higher grade tends to be more painful than the preceding one, except for grade 5, which may be relatively painless. With grade 5, the cessation of pain corresponds with the membrane tearing, which immediately equalizes the pressure in the middle ear with the external environment. Use of this grading scheme facilitates communication when describing these injuries.
TABLE 77-3 Teed Grading System for Middle Ear Barotrauma
Grade | Description |
---|---|
0 | Symptoms without otologic findings |
1 | Erythema and mild retraction of the TM |
2 | Erythema of the TM with mild or spotty hemorrhage within the TM |
3 | Gross hemorrhage throughout the TM |
4 | Grade 3 changes plus gross hemorrhage within the middle ear (hemotympanum) |
5 | Free blood in middle ear plus perforation of the TM |
TM, Tympanic membrane.
Topical and oral decongestants are often used before diving to facilitate clearing the ears.153 Pseudoephedrine has been reported to reduce the incidence and severity of middle ear barotrauma in novice divers.27 The use of intranasal surfactant has been suggested to improve eustachian tube function to prevent middle ear barotrauma during repetitive diving.69 Divers are sometimes taught not to use decongestants before diving because of theoretical concern of the medication wearing off while diving and causing problems during ascent; however, no data support this concern, and the judicious use of oral or nasal decongestants can facilitate pressure equalization.
Inner Ear Barotrauma
A serious but relatively unusual form of aural barotrauma is inner ear barotrauma, causing labyrinthine window rupture. This is the most serious form of aural barotrauma because of possible injury to the cochleovestibular system, which may lead to permanent deafness or vestibular dysfunction.72,81
Inner ear barotrauma results from rapid development of markedly different pressures between the middle and inner ear, such as may occur from an overly forceful Valsalva maneuver or an exceptionally rapid descent, during which middle ear pressure is not adequately equalized. During descent, the tympanic membrane is pressed inward, pushing the stapes against the oval window. The perilymph and endolymph are not compressible; the resulting increased pressure causes the round window to bulge outward. The diver may attempt a forceful Valsalva maneuver to equalize the middle ear; this will raise intracranial pressure, which is propagated through the perilymphatic duct to the inner ear, causing the round or oval window to rupture. Rupture of either window can also occur by a sudden increase in middle ear pressure by a forceful Valsalva. This pressure disequilibrium may cause several types of injury to the cochleovestibular apparatus, including hemorrhage within the inner ear; rupture of Reissner’s membrane, leading to mixing of endolymph and perilymph; fistulation of the oval or round window, with development of a perilymph leak; or a mixed injury involving any or all of these conditions.151 During ascent, the expanding middle ear gas may be forced through the perilymph fistula and enter either into the scala tympani or scala vestibule, which may damage cochlear or vestibular structures, leading to a permanent hearing loss.
Persons with inner ear barotrauma should be treated with bed rest (with the head elevated to 30 degrees), avoidance of any strenuous activity or any straining that can lead to increased intracranial pressure, and symptomatic measures as needed. There is a good prognosis for full recovery of hearing in 3 to 12 weeks. Labyrinthine window fistulas usually heal spontaneously, and data support conservative treatment initially.151 Deterioration of hearing, worsening of vestibular symptoms, or persistence of significant vestibular symptoms after a few days heralds the need for detailed otolaryngologic evaluation and possible surgical exploration and fistula closure. No consensus exists as to how long to wait before surgical intervention. If a perilymph fistula is suspected, it has been recommended to explore the ear surgically as soon as possible or if symptomatic after 24 hours.21 Patients with a tear in Reissner’s membrane have manifestations similar to those with inner ear hemorrhage, although there will be persistent localized sensorineural hearing loss commensurate with the area of membrane tear. Management is the same.
Dental Barotrauma
Barodontalgia may be caused by an array of dental conditions, including caries, defective restorations, oral tissue lacerations, recent extractions, periodontal abscesses, pulpal or apical lesions or cysts, and endodontal (root canal) therapy.157 If a pocket of trapped air remains at sea level pressure while ambient pressure increases during descent, the tooth can implode or the cavity fill with blood. Conversely, air that is forced into a tooth during descent can expand during ascent, causing the tooth to explode. To prevent barodontalgia, a diver probably should wait at least 24 hours after dental treatment (including fillings) before diving.
Lung Squeeze
In 1968, Schaefer and associates reported that breath-hold divers pool their blood centrally, accumulating a central volume increase of as much as 1047 mL at 27.4 msw (90 fsw).168 If it is assumed that this adjustment in pulmonary blood volume reduces the RV, then theoretically, it should be possible for the diver with a TLV of 6000 mL to breath-hold to 6000/(1200 − 1047 = 153), or almost 40 ATA. Although deep breath-hold dives seem to support the beneficial effect of central pooling of blood, cases of lung squeeze continue to occur at much shallower depths. The exact pathophysiology of this condition remains unclear. Fortunately, it occurs very infrequently.
Underwater Blast Injury
Barotrauma can be caused by underwater explosions. Shock waves from a blast are propagated farther in the dense medium of water than in air.41 Underwater explosions may result from ordnance or ignition of explosive gases during cutting or welding operations.
Underwater blasts can cause serious injuries to divers. Air-containing body cavities such as the lungs, intestines, ears, and sinuses are most vulnerable. Pneumothorax, pneumomediastinum, and air embolism may result from laceration of the lungs and pleura.102 There may be intestinal perforation, subserosals hemorrhage, and subsequent peritonitis. The occurrence of blast-related air embolism at depth, which worsens with ascent to the surface, requires treatment by recompression, if possible. Otherwise, management of underwater blast injuries is the same as for terrestrial blast injury.
Barotrauma of Ascent
Reverse Sinus or Ear Barotrauma (Reverse Squeeze)
The sinuses and ears are subject to barotrauma during ascent as well as descent. As the ambient pressure drops, the volume of the gas within the sinus or ear cavities expands, causing pain and tissue damage if not released. Gas pressure can exceed intravascular pressure in adjacent tissue, causing local ischemia. In the sinuses, a cyst or polyp can act as a one-way valve; air enters the sinus as the diver descends but cannot escape as the diver ascends. Fainting underwater as a result of frontal sinus pain has been reported.83
Alternobaric Vertigo
An unusual type of aural barotrauma is alternobaric vertigo (ABV). This usually occurs with ascent and is caused by sudden development of unequal middle ear pressure, which causes asymmetric vestibular stimulation and resultant pronounced vertigo.130 Vertigo, nausea, and vomiting may occur as the diver ascends. Although usually only transient and requiring no treatment, ABV may precipitate a panic response, leading to near drowning, pulmonary barotrauma, and resultant air embolism; however, the incidence of diving injuries due to ABV is unknown. Rarely, alternobaric vertigo lasts for several hours or days, in which case it should be treated symptomatically after excluding inner ear barotrauma. Diving should be avoided when middle ear equalization is compromised.
Alternobaric Facial Palsy
The seventh cranial nerve courses through the middle ear and mastoid process via a bony channel. Parts of the nerve may be directly exposed to middle ear pressures through a defect in the canal wall. During ascent, if the eustachian tube mucosa is swollen due to irritation, infection, or allergy, the middle ear pressure may exceed the capillary pressure of the facial nerve and cause an ischemic neuropraxia.71,104,139 The diver may complain of ear fullness and pain after surfacing, along with dysesthesias of the face and tongue. The diver is unable to close the eye on the affected side, and the mouth may be affected. Otoscopy reveals a bulging tympanic membrane.
Gastrointestinal Barotrauma
Because the intestines are pliable, contraction of intraluminal bowel gas during descent does not cause barotrauma. In unusual situations, however, expanding gas can become trapped in the gastrointestinal tract during ascent and cause gastrointestinal barotrauma, which is also known as aerogastralgia.45,129 This infrequent condition has been noted most often in novice divers, who are more prone to aerophagia; in divers who repeatedly perform the Valsalva maneuver in the head-down position, which may force air into the stomach, or those who chew gum while diving; and in divers who consume large quantities of carbonated beverages or legumes shortly before diving.
The physical examination of a diver having symptoms of gastrointestinal barotrauma is usually normal because the condition typically resolves by the time medical care is obtained. However, abdominal distention, tympany, and abdominal tenderness may be found. In an extreme case, there may even be signs of cardiovascular compromise as a result of obstruction of venous return.73 Gastrointestinal barotrauma can lead to pneumoperitoneum.120
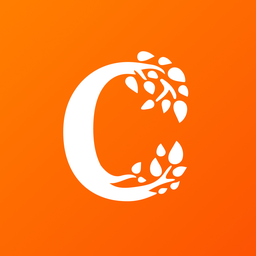
Full access? Get Clinical Tree
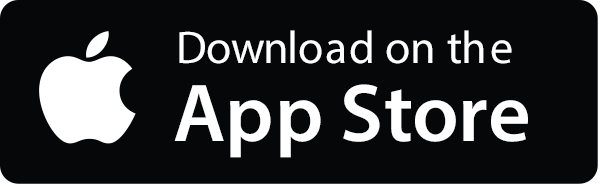
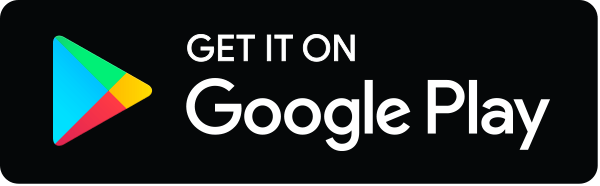