Developmental Aspects of Nociception
Suellen M. Walker
Although the International Association for the Study of Pain (IASP) definition of pain is based on the individual’s report of “a sensory and emotional experience,” it is also noted that “the inability to communicate verbally does not negate the possibility that an individual is experiencing pain and is in need of appropriate pain-relieving treatment” (1). This is crucially important in the management of pain in neonates, infants, and young children who are unable to directly describe their experience of pain, although their behavioral and physiologic response to pain may be considered a form of “self-report.” The assessment of pain is more difficult at these ages (2), but this does not lessen the need to adequately manage pain, not only for humanitarian reasons, but also because inadequately treated pain can have deleterious short- and long-term effects (3,4,5).
The immature nervous system responds to pain, injury, and analgesia very differently from the mature one. Activity within sensory pathways is required for normal development, but abnormal or excessive activity related to pain and injury during the neonatal period may alter normal development and produce persistent changes (6,7,8). Advances in neonatal and pediatric pain management are critically dependent on improved understanding of postnatal changes in: (a) nociceptive processing, (b) effects of different forms of injury, and (c) pharmacokinetic and pharmacodynamic responses to analgesic interventions (9,10).
The rat pup is born at a relatively immature stage and is an established model for investigation of developmental changes. Data collected from human foetal tissue and rat pups show a comparable pattern of progression throughout development, and approximate age correlations can be made across the two species (11). The development of peripheral and spinal cord somatosensory function in the rat from the embryonic day 10 (E10) to birth (E21.5) correlates with the first 24 postconceptional weeks of human gestation, and the first postnatal week in the rat pup corresponds to the later stages of human development from 24 weeks until full-term birth at 40 postconceptional weeks. Rat pups are weaned around the 21st postnatal day (P21) and by this age may be considered developmentally comparable to human adolescents (11,12). Rather than representing absolute correlations, these time lines provide a framework for assessing progressive changes in function throughout development.
This chapter outlines laboratory studies of nociceptive processing throughout postnatal development. More detailed descriptions can be found in recent reviews (13,14) and chapters (15,16). The response to different forms of injury throughout postnatal development will be described and, where possible, findings from laboratory and clinical findings will be compared. Finally, the developmental pharmacodynamics of common regional analgesics will be outlined.
Nociceptive Processing
During the first weeks and months of life, significant functional and structural changes in the developing nervous system influence nociceptive transmission. The expression of a number of molecules and channels involved in nociception are developmentally regulated, changes in the distribution and density of many important receptors occur, and the levels and effects of several neurotransmitters alter significantly during the postnatal period (13,14,17).
Peripherally Mediated Responses
The cell bodies of peripheral sensory neurons are located in the dorsal root ganglia (DRG) and send axons both peripherally to innervate target organs such as skin and centrally to synapse within the spinal cord. Large A-fibers innervate the skin before C-fibers, but by birth in the rat and the second trimester in man, sensory fibers are distributed to all body regions (15), and calcitonin gene-related peptide (CGRP) is being expressed in the skin (18). Peripheral cutaneous receptors are capable of responding to a range of stimuli from early development. In the rat pup, recordings from foetal (E16–E20) (19) and neonatal (P0–P14) (20) DRGs identified classes of sensory fibers responding to a similar degree and range of stimuli as adults (i.e., light touch or pressure, noxious pinch, heat, and chemical stimuli). Many different receptors mediate responses to peripheral stimuli, but the developmental profile of relatively few have been investigated. The transient receptor potential vanilloid-1 (TRPV1) receptor is activated by capsaicin, protons and thermal stimuli (>43°C), and the TRP ankyrin (TRPA1) receptor responds to pungent compounds such as mustard oil, and possibly noxious cold (21,22). Both TRPA1 and TRPV1 receptors are functional from early development, as primary afferent responses to mustard oil can be observed in fetal (E17–E20) and neonatal rat DRG (19,20), and initial responses to capsaicin are qualitatively similar in cultured DRG cells from neonatal (P0/1) and adult rats (23). TRPV1 nerve terminals are present in cutaneous structures early in development (24), and substance P-IR fibers are present in the skin, sciatic nerve, and dorsal horn at P1 (25). The sensory neuron-specific tetrodotoxin-resistant sodium channels (Na)v1.8 and Nav 1.9 are expressed on C-fibers at birth and reach adult levels by P7 (26).
Pain Transmission in the Spinal Cord
Afferent Input
In the adult, C-fiber polymodal nociceptors project to the superficial dorsal horn (lamina I and II), while larger myelinated Aβ-fibers, which subserve light touch and pressure, project to deeper layers of the dorsal horn (lamina III and IV). However, during development, the functional and anatomic relationships between C- and A-fibers change, leading to age-related changes in the processing of sensory inputs. Aβ-fibers enter the cord earlier than C-fibers, initially project throughout lamina I to V of the dorsal horn, and then gradually withdraw to the adult pattern of distribution in lamina III to IV over the first 3 postnatal weeks (18,27,28,29). A-fibers form synaptic contacts in the superficial laminae in the neonate (30), and A-fiber stimulation evokes postsynaptic spikes and sensitization in dorsal horn cells in the first postnatal week (31,32). As C-fiber function matures with increasing postnatal age, a progressive reduction occurs in A-fiber input and increase in C-fiber input (33,34). Although the intrinsic excitability of superficial dorsal horn neurons is stable throughout development (35), changes in synaptic inputs to these cells result in marked changes in the response to peripheral stimuli throughout development. During the initial period of A- and C-fiber overlap, receptive field sizes of dorsal horn neurons are large, which increases the degree of central activation following stimuli from a given area of peripheral tissue (31,36,37). In addition, the mechanical thresholds of individual dorsal horn sensory neurons are lower at P3 (37), thus increasing the central response to less intense stimuli in early development.
The response of dorsal horn neurons to C-fiber stimulation also varies with postnatal age. Functional synapses between TRPV1-expressing nociceptors and dorsal horn neurons can be identified in spinal cord slice preparations from early development. Initially, these synapses may lack the ability to synchronously release large amounts of transmitter, but a significant enhancement of effect arises from P5 to P10 (38). Extracellular recordings from dorsal horn cells reveal that C-fiber
electrical stimulation does not evoke postsynaptic responses in the first postnatal week (31,39). However by P10, cells in superficial laminae respond to a C-fiber stimulus, and repetitive C-fiber stimulation produces “wind-up” (31,32,36).
electrical stimulation does not evoke postsynaptic responses in the first postnatal week (31,39). However by P10, cells in superficial laminae respond to a C-fiber stimulus, and repetitive C-fiber stimulation produces “wind-up” (31,32,36).
Excitatory Modulation
The balance between excitatory and inhibitory neurotransmission in the dorsal horn alters during postnatal development (14). Enhanced excitatory mechanisms are important for activity-dependent changes during development (6), and there is a tendency for more delayed development of inhibitory mechanisms (40).
Developmental changes in the distribution and subunit composition of glutamate receptors in the spinal cord contribute to the increased excitability of the neonatal spinal dorsal horn (14). α-Amino-3-hydroxy-5-methyl-4-isoxazolepropionic acid (AMPA), kainate, and N-methyl-D-aspartate (NMDA) receptors are activated by glutamate to produce inward cation currents and depolarization of the postsynaptic cell. Binding sites for these receptors are initially higher in density and distributed more widely throughout the dorsal horn (41,42). The AMPA subunits GluR1, -2, and -4 are more highly expressed in the neonate, and increased GluR2 may increase the calcium permeability of the receptor (43,44,45). The subunits of the NMDA receptor are arranged in a tetrameric fashion, with two NR1 units and combinations of NR2A-D and/or NR3A-B. Subunit composition changes during development, with the NR2D subunit being highly expressed in neonatal cord (14). The affinity of the receptors for NMDA and the NMDA-induced increase in calcium influx are higher in substantia gelatinosa neurons in the first postnatal week (46). Glutamatergic synapses are functional from birth (38,47), but many synapses present in early development contain NMDA receptors exclusively (approximately 20%) (48). These pure NMDA receptors have been considered to be “silent” as they are not colocalized with AMPA receptors (49), but repetitive stimulation can drive action potential firing and affect neuron excitability in the absence of AMPA (47,50).
Inhibitory Modulation
Inhibitory mechanisms may not be fully mature in early life. Fast inhibitory transmission is mediated by γ-aminobutyric acid (GABA) and glycine receptors, both of which undergo developmental changes in subunit expression that influence channel kinetics (14). In the hippocampus, GABA initially produces depolarizing rather than hyperpolarizing currents, as the intracellular chloride concentration remains high until the potassium-chloride cotransporter KCC2 is upregulated later in development (51,52). However, GABAergic inhibition may mature earlier in the spinal cord. In dorsal horn slices, GABA-produced depolarization in a proportion of cells at P0 to P2, but was insufficient to produce action potentials, and by P6 to P7, only the adult pattern of hyperpolarizing responses was recorded. Glycinergic miniature inhibitory postsynaptic currents (IPSCs) could not be demonstrated at birth, and remained less frequent than GABAergic currents for the first 2 postnatal weeks (40). Application of the GABAA-antagonist gabazine to the spinal cord in vivo produced a similar degree of increased firing at P3 and P21, suggesting that intrinsic spinal GABAergic inhibition is functional during early life (53). Although GABA does not appear to have significant direct excitatory effects in the spinal cord, the overall response is also influenced by descending inputs. When the spinal cord is intact, facilitatory GABA-mediated effects on reflex responses can be identified in pups at P3. Following spinal cord transection, inhibitory GABA effects, as seen in older animals and in isolated spinal cord preparations, predominate. This suggests that modulation of GABA-mediated transmission by descending nociceptive pathways has important effects on the overall response, and these change throughout early development (54).
The development of interneurons in the superficial dorsal horn, some of which will be inhibitory, lags behind that of projection neurons (55). Descending inhibition of dorsal horn cell responses by stimulation of the dorsolateral funiculus is not present until P10 to P12, and until P22 to P24 is only activated by high-intensity stimulation (56). Diffuse noxious inhibitory controls are not functional in the first 2 postnatal weeks (57), and stimulus-produced analgesia from the periaqueductal gray is not apparent until P21 (58). Therefore, delayed maturation of interneuronal and descending inhibitory mechanisms may further shift the balance toward excitatory responses in the neonatal spinal cord.
The Withdrawal Reflex
Withdrawal reflexes are important models for the investigation of nociceptive processing at all ages, in both laboratory and clinical studies (59,60). Changes in the properties of the withdrawal reflex during development allow assessment of the changing sensitivity and selectivity of the neonatal nervous system to nociceptive stimuli (25,31). Measuring reflex thresholds allows quantification of responses to different forms of injury (61,62) and assessment of analgesic efficacy in preclinical studies (63,64,65,66). Similarly, changes in reflex thresholds in infants can provide additional objective evaluation of responses to injury and analgesia in clinical studies (67,68).
In the adult, the withdrawal reflex is selectively elicited by nociceptive stimuli (69). In early life, mechanical and thermal thresholds are lower, and the reflex response has greater amplitude, longer latency, and a higher degree of variability (14,25,70,71). The increase in withdrawal reflex thresholds with age reflects a gradual decrease in the excitability of spinal cord neurons, increased inhibitory input, and reorganization of sensory connections that reduce the size of the receptive field (31). Reflex responses are initially less organized, can be evoked by both noxious and innocuous stimuli, and may result in inappropriate generalized movements. Maturation of sensory and motor inputs, and an activity-dependent process that involves strengthening of appropriate connections and suppression of erroneous movements, leads to tuning of the receptive fields of each withdrawal reflex module. As a result, more specific motor responses develop that selectively move the stimulated area away from the stimulus (72,73,74).
In clinical studies, changes in reflex thresholds, receptive field size, and specificity of withdrawal responses have been demonstrated that correlate with the developmental pattern seen in laboratory investigations. The mechanical threshold of the hindlimb flexion withdrawal reflex is initially low and increases with postconceptional age (PCA) in preterm neonates, but is still well below adult levels at term (40 weeks PGA) (25,75,76). Electromyographic (EMG) responses of the biceps femoris to mechanical, electrical, and noxious (heel stick for routine blood sampling) stimuli confirmed lower reflex thresholds in infants aged 28 to 42 weeks PGA, and there was good correlation between stimulus intensity and the amplitude of the reflex at all ages (77). Reflex movements are also less synchronized in premature neonates. The receptive field of the hindlimb reflex is large in early development, as withdrawal can be elicited by low-intensity stimuli over the whole limb at 27 weeks PGA. As age increases, withdrawal is more specifically produced by stimuli on the foot, and there occurs a gradient of increasing threshold from distal to proximal sites on the
limb (76). A mechanical stimulus (von Frey hair) applied perpendicular to the abdomen produces a brisk contraction of the ipsilateral abdominal musculature in infants (abdominal skin reflex). In preterm neonates, a more generalized response that includes hip flexion is evoked, but the incidence and degree of hip flexion decreases sharply from 30 to 42 weeks PGA (68). Erroneous reflex movements have also been demonstrated in clinical studies. In an adult, stimulation of the plantar surface of the heel produces plantar flexion of the toes and no response in tibialis anterior (which produces dorsiflexion of toes). However, in neonates aged 30 to 39.5 weeks PGA, low-intensity mechanical (von Frey hairs) or electrical stimuli on the plantar surface of the foot produced an EMG response in tibialis anterior (60). This leads to inappropriate movement toward the stimulus, which resolves with further maturation and tuning of the reflex response.
limb (76). A mechanical stimulus (von Frey hair) applied perpendicular to the abdomen produces a brisk contraction of the ipsilateral abdominal musculature in infants (abdominal skin reflex). In preterm neonates, a more generalized response that includes hip flexion is evoked, but the incidence and degree of hip flexion decreases sharply from 30 to 42 weeks PGA (68). Erroneous reflex movements have also been demonstrated in clinical studies. In an adult, stimulation of the plantar surface of the heel produces plantar flexion of the toes and no response in tibialis anterior (which produces dorsiflexion of toes). However, in neonates aged 30 to 39.5 weeks PGA, low-intensity mechanical (von Frey hairs) or electrical stimuli on the plantar surface of the foot produced an EMG response in tibialis anterior (60). This leads to inappropriate movement toward the stimulus, which resolves with further maturation and tuning of the reflex response.
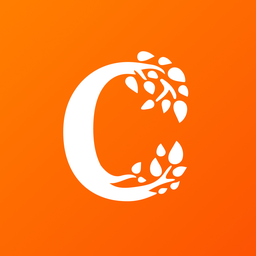
Full access? Get Clinical Tree
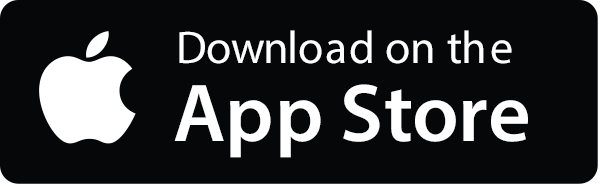
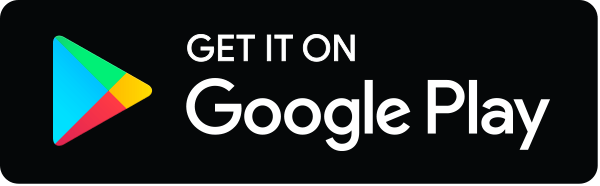