Chapter Outline
Why Monitor Depth of Anesthesia? 171
Currently Available Depth of Anesthesia Monitors 171
The Ideal Monitor 172
Assessment and Comparison of Awareness Monitors 172
Basic Principles of EEG Analysis 172
EEG Activity and Anesthetic Depth 173
Electromyographic (EMG) Component of Surface Voltages 173
EEG Processing Techniques 173
BIS Technology (Aspect Medical Systems) 174
Underlying Principle: Bispectral Analysis 175
S/5 Entropy Module (GE Healthcare) 177
Underlying Principle: Entropy 177
Narcotrend (MonitorTechnik) 179
Cerebral State Monitor (Danmeter) 180
Underlying Principle: Fuzzy Logic 180
SEDLine/Patient State Analyzer (Masimo) 181
SNAP II (Stryker) 181
AEP Monitor/2 (Danmeter) 182
Underlying Principle: Auditory Evoked Potentials 182
Emerging Technologies and Research 183
Conclusion 184
Why Monitor Depth of Anesthesia?
The problem of awareness during general anesthesia has become an increasingly prominent issue for both the anesthesiology community and the lay public. The Joint Commission issued a Sentinel Alert regarding awareness in 2004, and the American Society of Anesthesiologists (ASA) subsequently established a Task Force on Intraoperative Awareness that published a practice advisory regarding the use of brain function monitoring. This attention reflects the clinical significance of awareness because patients distressed by the recall of intraoperative events may develop severe psychological sequelae, including PTSD.
To address this problem, various devices have been developed to help assess the depth of anesthesia. It is worth noting that estimates of the incidence of awareness have ranged from 0.0068% in a study by Pollard et al to 1.0% in a study by Errando et al, making the identification of patients at risk for awareness and the reduction of this rare event a challenging task. There is a possibility that depth of anesthesia (DoA) monitors may also help anesthesia providers titrate medications, and help prevent oversedation, which may result in decreased morbidity and mortality. Regardless of the motivation for monitoring brain function, the anesthesia provider should consider the evidence regarding the clinical utility of the device with respect to the outcome of interest.
Currently Available Depth of Anesthesia Monitors
This chapter will discuss the following devices:
- ▪
Bispectral index monitors (Aspect Medical Systems)
- ▪
S/5 Entropy module (GE Healthcare)
- ▪
Narcotrend monitor (MonitorTechnik)
- ▪
Cerebral state monitor (Danmeter)
- ▪
SEDLine/patient state analyzer (Hospira)
- ▪
SNAP II monitor (Stryker)
- ▪
AEP Monitor/2 (Danmeter)
We will focus on these monitors because they represent the main devices that are currently used and researched and were the devices mentioned in the ASA Practice Advisory for Intraoperative Awareness and Brain Function Monitoring. Bowdle, Bruhn, and Jameson and Sloan have written reviews outlining the basic principles behind EEG monitoring and discuss the devices as they existed in 2006.
The Ideal Monitor
The ideal DoA monitor would (1) detect whether the patient is too lightly anesthetized and is at risk of awareness; (2) detect whether the patient is unnecessarily deeply anesthetized, and thus at risk for prolonged recovery; (3) work similarly across different patients; and (4) work similarly regardless of anesthetic modality and medications. Bruhn discusses the principles of validity and reliability of DoA monitors. The first two criteria relate to the concept of validity, which is the “accuracy” of the monitor in correctly determining the anesthetic depth. The latter two criteria relate to the concept of reliability, or “consistency” of the monitor in reporting the same result for a given depth of anesthesia.
The clinical utility of the device depends on how well it meets these criteria. By being accurate and consistent, a device should theoretically be able to demonstrate improvements in certain clinical end points, such as decreased awareness events or recovery times.
Assessment and Comparison of Awareness Monitors
This chapter will demonstrate the underlying computational approach of key monitors and examine the available evidence regarding the clinical utility of the devices. The intention is not to compare technologies with each other to determine the “best” equipment to use. Algorithms and technologies are continually updated, and there is much heterogeneity in studies in terms of patient populations and clinical end points. Generalizations made across various studies are thus often misleading, if not invalid. Sources purporting to provide product comparisons should be examined judiciously for both accuracy and bias. For example, an online “distributive evidence database” ( brainmonitor.doctorevidence.com ) offers to objectively synthesize and compare the outcome-oriented evidence for DoA monitors, but is funded by Aspect Medical Systems.
To discuss the merits of each DoA monitor, it is useful to have an easily communicated metric to describe the accuracy and reliability of the device. Sensitivity/specificity analyses or the comparison of likelihood ratios are two possible approaches. An alternate approach, adopted by the ASA Practice Advisory for Intraoperative Awareness and Brain Function Monitoring along with the majority of publications on DoA monitors, is to compare prediction probabilities.
Smith et al explains the concept of prediction probability (Pk) as applied to DoA monitors. Conceptually, Pk represents a measure of how well an index value (such as the BIS index) provided by the device can differentiate between different clinical states (e.g., response vs. no response to command, or levels of a graded sedation scale). If an index value were perfectly associated with the clinical state (i.e., a higher index value always represents a higher clinical level of arousal), the Pk value would be 1. If the index value were no better than chance in predicting a higher or lower clinical state, the Pk value would be 0.5.
The Pk is independent of scale, and does not make assumptions about the underlying distribution. It is thus commonly used for evaluating and comparing different monitors. The Pk essentially represents “criterion validity” (which is the agreement of the monitor with another instrument). However, the range of Pk values produced by various studies performed across different patients and anesthetic regimens are sometimes used as a measure of the reliability of the monitoring device.
Pk values are best used to compare devices in the same study. Comparisons between different studies may be confounded by differences in patient populations and anesthetic regimens. Pk values are also dependent on the clinical scale and assessment method used for comparison. For example, the Pk value for the prediction of loss of response to verbal command may be different from the Pk value for the prediction of loss of response to painful stimulus. The order of events is also important due to potential physiological hysteresis (i.e., loss of response during induction is not necessarily the reverse process of regaining response during emergence).
For purposes of evaluating a device’s ability to detect and prevent impending awareness, it may be best to use Pk values that assess some measure of response to stimulus upon emergence. It is also very important to note that Pk depends on the coarseness of the scale. A Pk value calculated using an “awake” versus “not awake” dichotomization would tend to have a higher value compared with a Pk calculated using multiple ordinal categories such as a 1 to 10 scale. This underscores the need to compare Pk values very cautiously.
In addition, Pks can be misleading if they are used as an indicator of the “believability” of a particular DoA monitor in predicting awareness in a given patient. Pks represent how well the monitor can predict a particular state across multiple patients; it does not offer direct information about the significance of a particular index value in a given patient. Index values must always be used as one piece of information that helps modify the individual pretest probability of awareness as determined by the clinician.
Basic Principles of EEG Analysis
In 1937, Gibbs et al published a study on the relationship between electroencephalography (EEG) patterns and drug-induced changes in neuronal activity. The authors presciently recognized the potential application of EEG technology as a monitoring device, commenting:
“a practical application … might be the use of the electro-encephalogram as a measure of the depth of anesthesia during surgical operations. The anesthetist and surgeon could have before them on tape or screen a continuous record of the electrical activity of both heart and brain.”
The regular adoption of EEG as a monitoring technology can be facilitated by the development of computerized EEG processing systems to analyze complex waveform patterns. Most current awareness monitors similarly collect EEG information with frontal electrodes, but they differ significantly in how these EEG signals are processed to yield an index value representing the depth of anesthesia. Processing techniques unique to certain devices will be discussed in the device-specific section of this chapter. Rampil provides an excellent technical primer on the physiology of EEG physiology and monitoring, while Sigl and Chamoun provide a very clear explanation of the basic mathematics behind EEG signal analysis.
EEG Activity and Anesthetic Depth
Synaptic activity of cells in the cortex results in voltage changes that can be detected by electrodes placed on the scalp. There are various waveform patterns within certain frequency ranges, which correspond to various neurophysiological processes. These patterns are grouped into frequency bands called, in order of increasing frequency, δ-, -, α-, β-, and γ-waves. Each band exhibits certain changes under the influence of anesthetic agents. A review by Jameson et al provides a detailed description of these neurophysiological and EEG effects.
Delta waves (<3 Hz) have the slowest frequencies of the various frequency bands and are seen in deep sleep; they may result from extreme thalamic depression. Θ-waves (4 to 7 Hz) may similarly arise from the inhibition of thalamic pacemaker cells. Theta waves are readily seen in young children and become less prominent with age, although they are normally seen during sleep at any age. Δ- and -waves are often referred to as “slow waves.” α-Waves (8 to 12 Hz) are prominently seen in awake patients and are prominent over the vertex in a relaxed or sedated state. They are thought to reflect the cyclical activity of thalamic pacemaker cells when anesthetics decrease the inhibition of the thalamus by the nucleus reticularis. The pyramidal area of alpha wave prominence tends to move to more frontal regions with increased depth of anesthesia in a process called “anteriorization” or “frontal predominance.”
Beta waves (12 to 24 Hz) are present in the prefrontal regions, and presumably reflect thalamocortical pathways with higher frequencies than α-waves due to desynchronization of the thalamus by sensory stimuli. Beta waves are normally present during alert states and can increase during initial central nervous system depression due to disinhibitory effects. This “β-activation” can be seen in Figure 12–1 . β activity is most prominent in the frontal regions and tends to move posteriorly with increased depth of anesthesia.

Gamma waves, also known as β 2 -bands, are of a higher frequency range (25 to 50 Hz) and may play a role in sensory processing and perception. Recent evidence suggests that organized γ activity may be essential for consciousness and interrupted during anesthesia. The EEG of an alert individual mostly consists of higher frequency α- and β-waves. With the addition of anesthetic agents, the overall amplitude of the EEG waveform initially increases as the EEG is synchronized in the 8 to 10 Hz range, and subsequently decreases with increased anesthetic depth. Similarly, the predominant frequencies initially increase during the early stages of anesthesia, after which the EEG shifts to lower frequency – and δ-waves.
With sufficiently deep anesthetic states (often minimal alveolar concentration [MAC] values of 1.5 or higher ), the EEG demonstrates a bilateral pattern of slow and mixed waves. A pattern of high amplitude activity (bursts) upon a flat baseline (suppression) is called “burst suppression.” A burst suppression ratio, comparing the percent duration of suppression, is often calculated. Burst suppression can also be seen with brain-injured conditions, such as postischemic states. Further deepening of the anesthetic state results in no discernable voltage changes (isoelectricity) ( Figure 12–1 ).
Electromyography (EMG) Component of Surface Voltages
Electrodes placed on the forehead may pick up voltage changes resulting from frontal facial muscle activity. This electromyographic (EMG) component can have a significant influence on the EEG recordings and may be either treated as an artifact or as a useful signal, depending on the particular DoA monitor. The band from 35 to 127 Hz, which may reflect muscle activity, was found in one study to provide the best performance for detecting awareness, but there is no clear agreement about the overall benefit of including EMG activity in EEG algorithms.
EEG Processing Techniques
The EEG can be analyzed in either the time domain or the frequency domain. Many of the improvements in DoA monitoring have resulted from the ability to process the EEG in the frequency domain. In the time domain, voltage changes in the EEG are plotted against time. Burst suppression is identified in the time domain. There are other time-domain techniques, such as the calculation of zero crossing frequency (ZXF), which is the number of times the EEG crosses the baseline, or a periodic analysis, which calculates the time between two consecutive minima of the waveform. Although these metrics are useful parameters in limited circumstances, complex signals cannot be fully analyzed by solely using time domain-based methods.
The advancement of microprocessing over the past few decades enabled fast calculations of Fourier transformations, which enable analysis of the frequency domain, wherein the frequencies of component signals present in the EEG are compared with the degree to which these frequencies are present. The general premise of EEG-based DoA monitors is that changes in EEG patterns can be detected, through time and frequency domain calculations, to provide an estimation of the level of consciousness.
Fourier Transformation and the Power Spectrum
Fourier analysis (also called spectral analysis) is based on the concept that complex waveforms can be approximated as a sum of sinusoids of various frequencies, amplitudes (power), and phase relationships, as shown in Figure 12–2 . A discrete Fourier transformation (DFT) converts a sample of periodic brain waves from the “time domain” to the “frequency domain” and “phase domain.” A fast Fourier transform (FFT) is an algorithm that very efficiently performs DFTs. Applied to an EEG, an FFT takes as an input the raw EEG waveform measured over time, and outputs the frequencies and phase of the component waves of the raw waveform. (A loose analogy for the FFT would be a prism separating out white light into individual colors of various wavelengths.)

The information about the frequency domain is often presented as a graph of power (the magnitude of the frequency contribution) versus frequency. The resulting “power spectrum” thus provides a measure of the extent to which various frequencies (corresponding to α-, β-, γ-waves, etc.) are present in the EEG.
Median Frequency and Spectral Edge Frequency
Early frequency-based analysis included simple parameters such as the peak frequency, the median frequency, or the spectral edge frequency. The peak frequency is often the frequency with the greatest power in a given wave region. The median frequency is the frequency that divides the power in half, and the spectral edge frequency is calculated as the frequency below which the majority (often, 90% or 95%) of the power lies ( Figure 12–3 ).

Nonlinearity and Phase Coupling
The cerebral cortex can be thought of as a system in which sinusoidal inputs (from neuronal triggers such as thalamic pacemakers) are entered into a “system” which outputs a certain EEG signal. Examination of the power spectrum alone provides information about the power and frequency, but not the phase relationships of the inputs. In a “linear” system, the output represents simple addition of each input, and the phase (time offset of the sinusoid) of the input does not matter. The neuronal system is complex, however, and should be thought of as a non linear system. As consequence of non linearity, the phase of the output signal depends on the phases of the input signals. The phases are then considered to be “phase coupled.”
As the degree of phase-coupling of various EEG frequency components is thought to reflect complex neurophysiological changes associated with various levels of consciousness, DoA monitors can use this phase coupling information to help predict the depth of anesthesia.
BIS Technology (Aspect Medical Systems)
Bispectral (BIS) technology is based upon an empirically determined proprietary algorithm developed by Aspect Medical Systems; it was approved by the U.S. Food and Drug Administration in 1996. It converts the recordings from a frontal EEG to a single number, the BIS index, which ranges from 0 (isoelectric) to 100 (awake) and represents the level of consciousness by the patient. The BIS system is the most widely used DoA monitor in the United States. According to information provided on the manufacturer’s website as of June, 2009, the BIS platform is installed in 75% of U.S. operating rooms, and 19% of procedures requiring general anesthesia or deep sedation use the BIS monitor. As such, most of the research pertaining to DoA monitors has been done on the BIS. In 2006, a PubMed search for the phrase “bispectral index” yielded 852 citations ; at the time of writing, the same search parameters revealed 1543 citations.
Following the earlier A-2000 and BIS VIEW monitoring platforms, the latest implementation of the BIS technology is the BIS VISTA system, which provides an option for bilateral monitoring. The BIS technology is available as a stand-alone system or can be incorporated into “BIS modules” for use with monitoring systems of external manufacturers ( Figure 12–4 ).

Underlying Principle: Bispectral Analysis
Bispectral analysis (also called two-dimensional spectral analysis) refers to the statistical process of quantifying quadratic nonlinearities of the system. The bispectrum, or bispectral value, represents the degree of phase coupling of two fundamental frequencies and a modulation frequency represented by the sum of the two frequencies ( Figure 12–5 ). A related parameter is the bicoherence, which is obtained by normalizing the bispectrum to a 0% to 100% range, to reflect the effect of relative amplitudes of the component signals. Part of the BIS algorithm calculates the logarithm of the ratio of the sum of bispectrum peaks in the range from 0.5 to 47 Hz to the sum of the peaks from 40 to 47 Hz. This value, known as the “SyncFastSlow,” is one of the many parameters used to calculate the BIS index. A full explanation of the calculation of the bispectral value is beyond the scope of this chapter, but can be found in the primer by Sigl and Chamoun.

The overall premise behind bispectral analysis is that changes in the clinical state are represented by changes in phase coupling. The neurophysiological significance of a large bispectral value being generated by two particular frequencies is not clear, but may represent the degree of shared EEG pacemaker elements.
What It Measures
Single Zipprep electrodes were initially developed for use with the BIS monitor, but the more recent versions have up to four single-use proprietary electrodes (BIS-XP Quatro) embedded in a single sensor. More recently, the BIS Vista bilateral monitoring system features four-channel EEG monitoring with two channels of information obtained from either side of the forehead to allow for hemispheric comparisons.
Of note, one study in 2002 found that BIS values obtained using generic ECG electrodes (costing 10 cents each) and proprietary BIS electrodes (costing $10 to $20) had acceptable limits of agreement. A study in 2007 similarly found no statistical difference in the signal quality index obtained from ECG electrodes compared with an unspecified version of BIS electrodes. Cost-effectiveness analysis regarding the newest iterations of the BIS electrodes is not currently available.
What It Outputs
To be useful for the clinician, the information resulting from the bispectral analysis is converted into an easily interpretable and clinically meaningful value.
For the BIS monitor, this value is the “BIS index,” which is calculated using a proprietary algorithm. The formula was empirically developed by correlating clinically assessed depths of anesthesia with features of the EEG including information from the frequency domain (i.e., power spectrum, bispectrum, and bicoherence) and the time domain (burst-suppression analysis).
The BIS VISTA display shows the BIS index value and trend and the raw EEG waveform and EMG indicator ( Figure 12–6 ).

The BIS bilateral system provides the option of a density spectral array (DSA) to be displayed ( Figure 12–7 ). This displays the trend of the power spectrum from each hemisphere simultaneously.

Clinical Utility
Although one study suggested that bispectral analysis does not give any additional information beyond information provided by the power spectrum, it is nonetheless worth examining the large body of literature regarding the clinical utility of the device. Of note, of the devices mentioned in this chapter, only the BIS monitor has been subjected to large-scale randomized clinical trials to assess whether usage of the monitor decreases the incidence of awareness. Despite the many studies to date, much remains controversial with respect to the clinical benefits of BIS monitors.
It is important to bear in mind that the BIS algorithm was empirically developed by the analysis of EEG effects of commonly used anesthetics such as propofol, midazolam, and isoflurane. The BIS index thus does not necessarily accurately represent the effects of all drugs. For example, the BIS Index has been shown to be unchanged upon administration of nitrous oxide, while ketamine has been shown to increase the BIS value. Although the traditional belief was that BIS indices do not accurately reflect xenon, a recent study using single-agent xenon anesthesia demonstrated that while the BIS index showed a delay to detect loss of response during induction, the BIS monitor was able to distinguish between consciousness and unconsciousness during steady state anesthesia.
Also, the BIS index may have lower values in patients with neurological conditions, such as cerebral ischemia, dementia, and severe hypoglycemia. The probability of explicit recall decreases below a BIS index of 70 and becomes extremely small below 60. Persistent BIS indices above 60 thus conservatively indicate that the patient may be at risk for intraoperative awareness, while levels below 40 may indicate unnecessarily excessive anesthetic administration. Although the generally accepted target range is 40 to 60, a recent study suggested that in opioid-heavy anesthetic cases, targeting a BIS value less than 60 may “result in an unnecessarily deep anesthetic state.”
Awareness-Related Outcomes
Given the low incidence of awareness, studies examining the impact of BIS monitoring on awareness reduction require large sample sizes. In 2004, Ekman et al performed a prospective cohort study of 4945 surgical patients who were monitored with the BIS, using a protocol to keep the BIS index between 40 and 60. Using a historic control, the study found a statistically significant reduction in awareness (0.04% in the BIS group compared with 0.18% in the control group).
In the same year, Myles et al conducted the “B-Aware” study, randomizing 2643 patients at high risk of awareness to either routine care or BIS-guided anesthesia. Two of the 1225 patients assigned to the BIS-guided anesthesia group and 11 patients of the routine care group reported awareness, resulting in a statistically significant reduction in the relative risk of awareness by 82% and a number needed to treat (NNT) of 138 patients. The study did not show any significant difference in death rate, satisfaction, or postoperative complications.
In early 2008 a highly publicized study (the “B-Unaware” study) was published by Avidan et al. Two thousand patients were randomly assigned to BIS-guided anesthesia (targeting a BIS value of 40 to 60), or to a protocol based on end-tidal anesthetic gas (ETAG), for which the target ETAG range was 0.7 to 1.3 MAC. Two cases of definite awareness were found in each group (with 967 patients and 974 patients in the BIS and ETAG groups, respectively), for an absolute difference of 0% (95% CI −0.56% to 0.57%).
There has been considerable controversy over the conflicting conclusions given by the B-Aware and the B-Unaware study. In a letter to the editor, representatives of Aspect have criticized the B-Unaware for an inadequate sample size calculation. However, appropriate interpretation of confidence intervals may be more meaningful than posthoc criticisms of power analysis. Regardless of the statistical power of the study, the results show that within the 95% confidence interval, the “best case” scenario in favor of the BIS is a −0.56% difference in the rate of awareness. Another criticism is that the choice of the ETAG-based alert system as a standard of comparison does not reflect a routine “standard of care” in most practices. The conclusion drawn from the study by the B-Unaware trial should be that the BIS monitor does not appear to provide an additional benefit over ETAG monitoring.
Summarizing the available evidence, a 2007 Cochrane review examined 20 studies with 2056 participants, and concluded that BIS-guided anesthesia significantly reduced the incidence of intraoperative recall awareness in surgical patients with high risk of awareness (OR 0.20, 95% CI 0.05 to 0.79). In contrast, the 2006 ASA Practice advisory cautioned that despite the results of the B-Aware trial, “there is insufficient evidence to justify a standard, guideline, or absolute requirement that (depth of anesthesia) devices be used to reduce the occurrence of intraoperative awareness in high-risk patients undergoing general anesthesia.”
The aforementioned studies have focused on BIS usage in a population at high risk for awareness, and there is no current evidence regarding whether or not BIS monitoring helps reduce the incidence of awareness in routine cases.
Secondary Outcomes
Monk provides a review of the evidence for improved patient outcomes with BIS usage and concludes that EEG monitoring appears to provide a “modest reduction in intraoperative primary anesthetic agent dosing of approximately 20% to 25%,” which was linked to other benefits such as a reduction in early recovery time, postoperative nausea and vomiting, and possibly long-term outcomes following major surgery. Similarly, the 2007 Cochrane review found that the use of BIS statistically significantly reduced propofol requirements by 1.30 mg/kg/hr and volatile anesthetic use by 0.17 MAC. BIS also reduced the recovery times, including time for eye opening, response to verbal command, time to extubation, and orientation by 2 to 3 minutes. The duration of postanesthesia care unit stay decreased by 6.8 minutes but time to home readiness remained the same. The review concluded that “anesthesia guided by BIS within the recommended range (40 to 60) could improve anesthetic delivery and postoperative recovery from relatively deep anesthesia.” However, the ASA Task Force on Intraoperative Awareness suggested that “brain function monitoring is not routinely indicated for patients undergoing general anesthesia, either to reduce the frequency of intraoperative awareness or to monitor depth of anesthesia.”
In addition, a study by Monk et al found that prolonged deep anesthesia (defined as the time during which the BIS value was less than 45) was a significant independent risk factor for 1-year postoperative mortality. However, a recent follow-up study by Lindholm et al found that the relationship between either 1- or 2-year mortality and the time of deep anesthesia was not significant when preexisting malignancy was included as a covariate. Further work is required on the relationship of anesthetic depth, malignancy, and mortality.
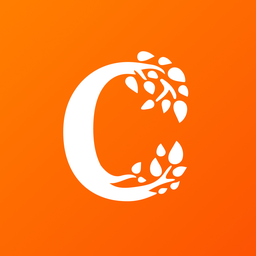
Full access? Get Clinical Tree
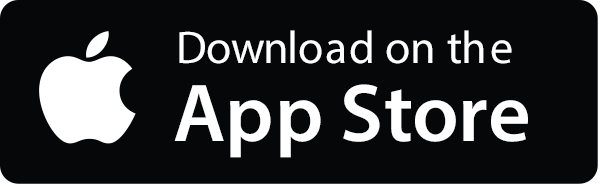
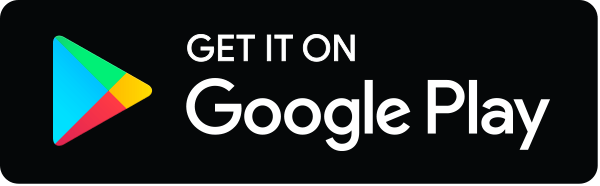