Chapter 70 Dehydration, Rehydration,* and Hyperhydration
Introduction and Definition of Terms
Body fluid balance is controlled by both physiologic and behavioral actions.44,84 However, when there is lack of fluid availability, exposure to extreme environments, or illness, inability to maintain fluid balance can seriously jeopardize health and the ability to perform.84 This chapter presents an overview of topics surrounding hydration, dehydration, and rehydration. The terms euhydration, hypohydration, and hyperhydration will be used. Euhydration defines a “normal,” narrow fluctuation in body water content, whereas the terms hypohydration and hyperhydration define, respectively, a general deficit (hypohydration) and surfeit (hyperhydration) in body water content beyond normal. The term dehydration specifically defines the condition of hypertonic hypovolemia brought about by the net loss of hypotonic body fluids. Isotonic or hypotonic hypovolemia, manifest by large losses of solute and water, is defined simply as hypovolemia.75,109 Table 70-1 lists the two principal forms of body water deficit and the physiology and particular circumstances associated with each form of deficit.
TABLE 70-1 Two Principal Forms of Body Water Deficit
Form | Physiology | Circumstances |
---|---|---|
Hypertonic hypovolemia | ||
Isotonic hypovolemia |
ECF, Extracellular fluid; ICF, intracellular fluid.
Body Water, Fluid Turnover, and Fluid Requirements
Total body water (TBW) is the principal chemical component of the human body and represents 50% to 70% of body mass7 for the average young adult male. It is regulated within ±0.2 to 0.5% of daily body mass.2,35 Body water is required to sustain the cardiovascular and thermoregulatory systems and to support cellular homeostasis. Although “normal” hydration is achieved with a wide range of water intakes by sedentary and active people across the life span, homeostasis of body water can be difficult to maintain when challenged by strenuous physical work, heat stress, or illness. Despite population variability in age, body composition, and physical fitness, it is important to note that variability in TBW is accounted for almost entirely by body composition, since lean body mass contains approximately 73% water and fat body mass consists of approximately 10% water.196 Trained athletes have relatively high TBW values by virtue of having a high muscle mass and low body fat. In contrast, obese individuals with the same body mass as their lean counterparts will have markedly smaller TBW volumes. Any absolute fluid deficit will have more severe consequences for individuals with a smaller TBW.
Daily water balance depends on the net difference between water gain and water loss.84 Approximately 5% to 10% of TBW is turned over daily152 via obligatory (nonexercise) fluid loss avenues. Water gain occurs from consumption (liquids and food) and production (metabolic water), whereas water losses occur from respiratory, gastrointestinal, renal, and sweat losses. Water loss in respiration is influenced by the inspired air and pulmonary ventilation. Of important note, the volume of metabolic water produced during cellular metabolism (approximately 0.13 g/kcal) is approximately equal to respiratory water losses (approximately 0.12 g/kcal),45,122 which results in water turnover with no net change in TBW. Gastrointestinal tract losses tend to be negligible (approximately 100 to 200 mL/day); however, certain illnesses, such as diarrhea, can lead to loss of large amounts of fluid and electrolytes. The ability to vary urine output represents the primary means to regulate net body water balance across a broad range of fluid intake volumes and losses from other avenues. Water losses in urine approximate 1 to 2 L/day. However, urine output volumes may be larger or smaller depending on daily fluid consumption and activity.84 Minimum outputs of approximately 20 mL/hr and maximal volumes of approximately 1000 mL/hr are possible.
Net body water balance (loss = gain) is regulated remarkably well day-to-day as a result of thirst and hunger, coupled with ad libitum access to food and beverages, which offset water losses.84 Although acute mismatches between fluid gain and loss may occur due to illness, environmental exposure, exercise, or physical work, it is a reproducible phenomenon that intakes are generally adequate to offset net loss from day to day.47 It is recognized, however, that after significant body water deficits like those associated with physical work or heat stress, many hours of rehydration and electrolyte consumption may be needed to reestablish body water balance.183 For example, if hypohydrated by more than about 4% of total body mass, it may take >24 hours to fully rehydrate via water and electrolyte replacement.4,129,138 While daily strenuous activity in a hot environment can result in mild water balance deficits even with unlimited access to food and fluids,9,183 adherence to recognized water intake guidance9,30,165 minimizes water deficits, as determined by daily body mass stability.35
An adequate intake (AI) for daily total water is 3.7 L and 2.7 L for adult males and females, respectively.84 Of these prescribed volumes, 20% of the AI for water is found in food eaten during meals and snacks and the remaining 80% (approximately 3 L for males and 2.2 L for females) can come from beverages of all types. Daily water intake, however, varies greatly for individuals and between groups. For example, the daily water needs of sedentary men are approximately 1.2 L to 2.5 L1,137 and increase to approximately 3.2 L if performing modest physical activity.76,79 Compared with sedentary adults, active adults who live in a warm environment are reported to have daily water needs of approximately 6 L,201 and highly active populations have been reported to have markedly higher values.162 Data are limited regarding fluid needs for women, but typically they exhibit lower daily water turnover rates than do their male counterparts. In general, fluid requirements vary based on an individual’s body size, activity level, and the environment in which he or she works, lives, or performs activity.
Hydration Assessment
Human hydration assessment is a key component for prevention and proper treatment of fluid and electrolyte imbalances.41,109,146 When fluids are limited, illness strikes, or there is exposure to extreme environments, cumulative fluid deficits can threaten homeostasis, health, and performance.109,165 Health is also threatened by fluid deficits, which can increase the risk of serious heat illness, and by fluid surfeits, which increase the risk of hyponatremia.28,123 In many clinical and most sports and wilderness medicine situations, hypertonic-hypovolemia occurs when there is net loss of hypotonic body fluids.41,109,165 However, substantial solute (electrolyte) can also be lost in situations where there is heavy work, and heat stress induces profuse sweating, during cold or high-altitude exposure, and in numerous illnesses and disorders (e.g., gastroenteritis, hyperemesis, diuretic treatment, dialysis) producing an isotonic or hypotonic-hypovolemia.41,109,165 An appreciation for the different types of body fluid losses that occur in response to illness, fluid restriction, or exposure to extreme environments is fundamental to proper hydration assessment41,109 (Table 70-1).
Most circumstances involving strenuous work in austere environments require formation and vaporization of sweat as a principal means of heat removal. Thus, when sweat losses result in a body water deficit, there is a predictable rise in extracellular tonicity, which modulates renal function and urine composition in accordance with the body water deficit.156 The basic principles of body fluid regulation thus provide the framework for using blood (osmolality, sodium, fluid regulatory hormones) and urine (osmolality, specific gravity, color) as principal body fluid hydration assessment measures. Similarly, because humans maintain a relatively stable total body water pool despite diverse factors that affect water requirements (e.g., climate, activity, dietary solute load),84 acute changes in body mass may be used to accurately measure dehydration across medical disciplines.14,35,195 Physical signs and symptoms (dizziness, headache, tachycardia, capillary refill time, sunken eyes, skin turgor) only manifest when fluid losses are severe and become debilitating.64,191 These findings are too nonspecific to be useful in athletic settings116 since they share symptoms indicative of other ailments (e.g., acute mountain sickness) and their use in assessment could lead to an incorrect diagnosis.
All hydration assessment methods vary greatly in applicability because of limitations such as the necessary circumstances for reliable measurement, principles of operation, cost and complexity.84,146 Table 70-2 provides the advantages and disadvantages of numerous approaches and should be consulted when deciding on the choice of hydration marker. Definitive hydration assessment requires monitoring of changes in hydration state.38,117 Although change can provide good diagnostic accuracy, it requires a valid baseline, control over confounding variables, and serial measures.38,117 Large population heterogeneity explains, in part, why there are presently few hydration status markers that display potential for high nosologic sensitivity from a more practical, single measure.38,103 Although Table 70-3 provides euhydration thresholds for the most useful of hydration assessment measures, they too require considerable methodologic control, expense, and analytical expertise to be of practical use for day-to-day hydration monitoring of athletic sojourners.
TABLE 70-2 Hydration Assessment Techniques Summary41
Technique | Advantages | Disadvantages |
---|---|---|
Complex Markers | ||
Total body water (dilution) | Accurate, reliable (gold standard) | Analytically complex, expensive, requires baseline |
Plasma osmolality | Accurate, reliable (gold standard) | Analytically complex, expensive, invasive |
Simple Markers | ||
Urine concentration | Easy, rapid, screening tool | Easily confounded, timing critical, frequency and color subjective |
Body mass | Easy, rapid, screening tool | Confounded by changes in body composition |
Other Markers | ||
Blood: | ||
Plasma volume Plasma sodium Fluid balance hormones | No advantages over osmolality (except hyponatremia detection for plasma sodium) | Analytically complex, expensive, invasive, multiple confounders |
Bioimpedance | Easy, rapid | Requires baseline, multiple confounders |
Saliva | Easy, rapid | Highly variable, immature marker, multiple confounders |
Physical signs | Easy, rapid | Too generalized, subjective |
Tilt test (orthostatic challenge) | Rapid | Highly variable, insensitive, requires tilt table or ability to stand |
Thirst | Positive symptomatology | Develops too late and is quenched too soon |
There is presently no scientific consensus for how to best assess hydration status in a field setting. However, in most field settings, the additive use of first morning body mass measurements in combination with some measure of first morning urine concentration and gross thirst perception provides a simple and inexpensive way to dichotomize euhydration from gross dehydration resulting from sweat loss and poor fluid intakes. This approach is represented using a Venn Diagram decision tool (Figure 70-1).41 It combines three of the simplest markers of hydration, including body mass (weight), urine, and thirst (WUT). No marker by itself provides enough evidence of dehydration, but the combination of any two simple self-assessment markers means dehydration is likely. The presence of all three makes dehydration very likely. The balance between science and simplicity in the choice of these measures for field hydration assessment is outlined below.
Urine Concentration
Urinalysis is a frequently used clinical measure to distinguish between normal and pathologic conditions. Urinary markers for dehydration include urine volume, urine specific gravity (USG), urine osmolality (UOsm) and urine color (UCol). Urine is a solution of water and various other substances. Thus, its concentration varies inversely with volume, which is reduced with dehydration. Urine output generally approximates 1 to 2 L/day but can be increased by an order of magnitude when consuming large volumes of fluid.84 This large capacity to vary urine output represents the primary avenue to regulate net body water balance across a broad range of fluid intake volumes and losses from other avenues. Whereas the quantification of urine volume is impractical on a daily basis, the quantitative (USG, UOsm) or qualitative (UCol) assessment of its concentration is far simpler. As a screening tool to dichotomize euhydration from dehydration, urine concentration (USG, UOsm, UCol) is a reliable assessment technique9,17,182 with reasonably definable thresholds.
In contrast, urine measures often correlate poorly with “gold standards” like plasma osmolality and fail to reliably track documented changes in body mass corresponding to acute dehydration and rehydration.97,151 It appears that changes in plasma osmolality that stimulate endocrine regulation of renal water and electrolyte reabsorption are delayed at the kidneys when there occur acute fluxes in body water.151 It is also likely that drink composition influences this response. Shirreffs and Maughan186 demonstrated that drinking large volumes of hypotonic fluids results in copious urine production long before euhydration is achieved. Urine concentration measurements can also be confounded by diet, which may explain large cross-cultural differences in urine osmolality.110 However, use of the first morning void following an overnight fast minimizes confounding influences and maximizes measurement reliability.9,182 Urinalysis of specific gravity, osmolality, and color can therefore be used to assess and distinguish euhydration from dehydration so long as the first morning void is used.
Inexpensive and easy-to-use commercial instruments are available for assessing USG and conductivity (osmolality equivalent)17,182; a urine color chart is also available.9 The simplest of these, color, is included in the Venn diagram. Under ideal circumstances, the urine (first morning) should be in a clean, clear vial or cup and the color assessed against a white background. Urine color can be compared against a urine color chart9 or assessed relative to the degree of darkness. Paler color urine (similar to lemonade) indicates adequate hydration and the darker yellow/brown the urine color (similar to apple juice), the greater degree of dehydration. Assessing urine that has been diluted in toilet water or while in mid-flow may alter the urine color. When in less than ideal conditions, urine in a urinal is less dilute and in the field, snow can provide a suitable background. Example photos of urine color with corresponding numeric color,9 USG, and urine osmolality values are presented in Figure 70-2.
Body Mass
Body mass is an often used measurement for rapid assessment of athlete hydration changes in both laboratory and field environments. Changes in acute hydration are calculated as the difference between pre- and postexercise body masses. The level of dehydration is best expressed as a percentage of starting body mass rather than as a percentage of TBW, since the latter ranges widely.84 Use of this technique implies that 1 g of lost mass is equivalent to 1 mL of lost water. As long as total body water loss is of interest, failure to account for carbon exchange represents the only small error (approximately 10%) in this assumption.39 Indeed, acute body mass changes (water) are frequently the standard against which the resolution of other hydration assessment parameters are compared in the laboratory. In fact, if proper controls are made, body mass changes can provide a more sensitive estimate of acute total body water changes than can repeat measurements by dilution methods.78
There is also evidence that body mass is a sufficiently stable physiologic parameter for potential daily fluid balance monitoring, even over longer periods (1 to 2 weeks) that include hard exercise and acute fluid flux.35,102 Young, healthy men undergoing daily exercise-heat stress maintain a stable first morning body mass as long as they make a conscious effort to replace exercise-induced sweat losses.35 Similarly, ad libitum intakes of food and fluid will balance sweat losses incurred with regular exercise, resulting in a stable daily body mass.102 Over longer time frames, changes in body composition (fat and lean mass) that occur with chronic energy imbalance are also reflected grossly as changes in body mass, thus limiting this technique. Clearly, if first morning body mass stability is used to monitor changes in hydration, it should be used in combination with another hydration assessment technique (urine concentration) to dissociate gross tissue losses from water losses if long-term hydration status is of interest.
Thirst
Although genuine thirst develops only after dehydration is present and is alleviated before euhydration is achieved,75,84 thirst is one of the only reliable subjective feelings reported by a person in response to fluid restriction.184 Plasma osmolality near 295 mmol/kg will produce an arginine vasopressin (AVP) level of around 5 pg/mL, which results in a maximal urine concentrating capacity.156 The average plasma osmolality at which thirst is stimulated above baseline is also approximately 295 mmol/kg.156 If we assume that a normal resting plasma osmolality of 285 becomes concentrated to 295, the ratio 285/295 multiplied by a normal 42 L total body water gives an estimated 40.5 L or 1.5 L total body water deficit, which is 2.1% dehydration for a 70-kg person. This is consistent with general observations of thirst insensitivity below a “threshold” fluid deficit (notion that thirst develops late). However, it is important to recognize that there is substantial individual variability in the plasma osmolality “set point” and the osmotic thresholds for AVP release and thirst perception.157 For example, Robertson reports as much as a 10-fold difference between individuals in the slope of the line relating AVP to plasma osmolality.157 Clearly, thirst is a qualitative tool for hydration assessment, but positive thirst symptoms coupled with at least one additional Venn Diagram marker suggests an increasing likelihood of dehydration.
Although plasma osmolality and total body water measurements are the hydration assessment measures for large-scale fluid needs,84 there is presently no consensus for using any one approach over another in a field or athletic setting. In most circumstances, the use of first morning body mass combined with some measure of first morning urine concentration (USG, urine osmolality, and/or color) offers simple assessment and allows ample sensitivity (low false negative) for detecting meaningful deviations in fluid balance (>2% body mass). This approach is represented using a Venn Diagram decision tool (see Figure 70-2).41 It combines three of the simplest markers of hydration, including weight, urine, and thirst (WUT). No marker by itself provides enough evidence of dehydration, but the combination of any two simple self-assessment markers means dehydration is likely, and the presence of all three makes dehydration very likely. In a field setting, where a scale may not be available for body weight measures, the combination of first morning urine color and thirst may provide a reasonable indication of the presence of dehydration.
Sweat and Sweat Prediction
Muscular contractions involved with activity/exercise produce metabolic heat that is transferred from the active muscles to blood and then the body core. Subsequent body temperature elevations elicit heat loss responses of increased skin blood flow and increased sweat secretion so that heat can be dissipated to the environment.173,175 Heat exchange between the skin and environment is governed by biophysical properties dictated by surrounding temperature, humidity and air motion, sky and ground radiation, and clothing.67 When ambient temperature is greater than or equal to skin temperature, evaporative heat loss accounts for all body cooling. Eccrine sweat glands secrete fluid onto the skin surface, permitting evaporative cooling when liquid is converted to water vapor. Sweat glands respond to thermal stress primarily through sympathetic cholinergic stimulation, with catecholamines having a smaller role in the sweat response.175 The rate of sweat evaporation depends on air movement and the water vapor pressure gradient between the skin and environment, so in still or moist air, sweat does not evaporate readily and collects on the skin. Sweat that drips from the body or clothing provides no cooling benefit. If secreted sweat drips from the body and is not evaporated, higher sweating will be needed to achieve the evaporative cooling requirements.36,173 Conversely, increased air motion (wind, movement velocity) will facilitate evaporation and minimize wasted (dripping) sweat.36 Sweat losses can vary widely and depend on the amount and intensity of physical activity and environmental conditions.71,180 In addition, a number of factors can alter sweat rates and ultimately fluid needs. Heat acclimatization results in higher and more sustained sweating rates.173,175 Similarly, aerobic exercise training has a modest effect on enhancing sweating rate responses.173,175 Wearing heavy or impermeable clothing or protective equipment can increase heat stress115 and sweat rate, but can limit evaporation of sweat and ultimately heat loss. Likewise, wearing heavy or impermeable clothing while exercising in cold weather can elicit unexpectedly high sweat rates,63 which can increase fluid needs. Conversely, factors such as wet skin (e.g., from high humidity) and dehydration can act to suppress the sweating rate response.173
Sweat is hypotonic relative to plasma and is typically one-half of plasma osmolality (approximately 145 mmol/kg vs. approximately 290 mmol/kg, respectively).46 Losses of electrolytes in sweat depend on total sweat losses (over a given period of time) and sweat electrolyte concentrations. Typical sweat sodium concentration averages approximately 35 mEq/L (range 10 to 70 mEq/L) and varies depending on genetic predisposition, diet, sweating rate, and heat acclimatization state.* Sweat concentration of potassium averages 5 mEq/L (range 3 to 15 mEq/L), calcium averages 1 mEq/L (range 0.3 to 2 mEq/L), magnesium averages 0.8 mEq/L (range 0.2 to 1.5 mEq/L), and chloride averages 30 mEq/L (range 5 to 60 mEq/L).22 Gender, maturation, or aging do not appear to have discernible effects on sweat electrolyte concentrations,120,130 although dehydration can increase the sweat concentrations of sodium and chloride.128 Sweat glands reabsorb sodium and chloride by active transport, but the ability to reabsorb these electrolytes does not increase proportionally with the sweating rate. As a result, the sodium and chloride concentrations of sweat increase as a function of sweating rate.5,47 Heat acclimatization improves the ability to reabsorb sodium and chloride; thus, heat-acclimatized individuals usually have lower sweat sodium concentrations (e.g., >50% reduction) for any given sweating rate.5
The ability to predict sweat losses is critical for calculating water needs, particularly for individuals who are exposed to heat stress. For large cohorts such as the U.S. military, fluid requirements in the field are based on water tables generated from existing sweat prediction equations. Sweat rates differ between various work activities and between individuals.173 Specifics on determining individual sweat rate and fluid requirements are covered later in the chapter in the fluid replacement recommendations section. Figure 70-384 depicts generalized modeling approximations for daily sweating rates as a function of daily metabolic rate (activity level) and air temperature. Metabolic rate and air temperature both have marked effects on water needs. In addition to air temperature, environmental factors such as relative humidity, air motion, solar load, and protective clothing influence heat strain and water needs.
Figure 70-3 shows approximations of water needs. The Shapiro equation180 has been used extensively to estimate sweating rates and calculate daily water needs. This model calculates sweat rate (Msw; expressed as g m−2 h−1) and thus fluid needs as the following:
where Ereq is the required evaporative cooling (in W/m2) and Emax is the maximal evaporative cooling capacity. Ereq is calculated from metabolic heat production, clothing heat transfer characteristics, and the environment. Emax is derived from the vapor transfer properties of the clothing worn and the environment. However, this equation has been shown to have limitations,40 in that it often overpredicts fluids needs when exercise is greater than 2 hours, when improved uniforms and body armor are worn, and when activity takes place in lower air temperatures and the activity is of high intensity. In response to these limitations, Gonzalez and colleagues71 developed a correction for the Shapiro equation in addition to a new prediction equation using independent data across a wide range of environmental conditions, metabolic rates, and environmental conditions. Figure 70-4, taken from Jay and associates,86 depicts the adjustment to the sweat loss values yielded by the corrected Shapiro equation derived by Gonzalez and co-workers.71 Future work in this area may be required to further increase the applicability of sweat rate prediction equations, in particular under conditions with variable solar loads, in lower air temperatures (approximately 15° C [59° F]), with clothing having low water vapor permeability or with specialized equipment (e.g., American football), and with individuals possessing greater body mass and surface areas.86 At present, use of such equations has been predominantly limited to the military. Future commercial applications of such equations to predict fluid needs by the general population in hiking/trekking/wilderness scenarios may be possible via commonly used devices, such as personal digital assistants (PDAs).
Physiologic Consequences of Dehydration
By virtue of tonicity and volume changes, dehydration has negative consequences on thermoregulation and performance. Dehydration is brought about by voluntary fluid restriction, insufficient rehydration following daily activity, or physical activity/exercise in the form of thermoregulatory sweating. The most common form of dehydration during exercise in the heat is that where water deficit occurs without proportionate sodium chloride loss.166 Individuals often start an exercise task with normal total body water and dehydrate over an extended duration; however, in some situations, an individual might initiate activity/exercise with a body water deficit because the interval between exercise sessions is inadequate or chronic fluid intake is insufficient to replace losses. During multiple-day treks or expeditions where individuals take part in prolonged daily sessions of activity/exercise, possibly in hot conditions, a fluid deficit may be carried from one activity/exercise session to the next or from one day to the next.70 In addition, individuals medicated with diuretics may be dehydrated prior to initiating exercise. Use of medications, such as acetazolamide taken prophylactically or while at altitude for acute mountain sickness, can have such an effect (and may increase risk for hyponatremia). This drug causes the kidneys to excrete bicarbonate, which acidifies the blood, increasing ventilation and blood O2 content; however, it also increases fluid and electrolyte losses. If large sodium chloride deficits occur during exercise, then the extracellular fluid volume contracts and causes “salt depletion dehydration.”
Dehydration increases physiologic strain as measured by core temperature, heart rate, and perceived exertion responses during exercise heat stress.166 The greater the body water deficit, the greater the increase in physiologic strain for a given exercise task.3,124,125,176 Dehydration can augment core temperature elevations during exercise in temperate26,136,170 as well as in hot environments.43,168,178 The typical reported core temperature augmentation with dehydration is an increase of 0.1° to 0.2° C (32.18° to 32.36° F) with each 1% of dehydration.167 The greater heat storage associated with dehydration is associated with a proportionate decrease in heat loss. Thus, decreased sweating rate (evaporative heat loss) as well as decreased cutaneous blood flow (dry heat loss) are responsible for greater heat storage observed during exercise when hypohydrated.59,60,134 The degree to which each of these mechanisms dissipates heat from the body depends on environmental conditions. However, both avenues of heat loss are unfavorably altered by dehydration.
When dehydrated, the sweating rate is lower for any given core temperature, and heat loss via evaporation is reduced.176 In addition, as dehydration increases, there is reduction in total body sweating rate at a given core temperature during exercise heat stress.176 During submaximal exercise with little or no thermal strain, dehydration results in increased heart rate and decreased stroke volume, and typically no change in cardiac output relative to euhydration levels.163,188 The addition of heat stress in combination with dehydration during exercise results in decreased blood volume, reducing central venous pressure and cardiac output,94,129 and creates competition between the central and peripheral circulation for limited blood volume.133,161 As body temperature increases during exercise, cutaneous vasodilation occurs and superficial veins become more compliant, decreasing venous resistance and pressure.161 A result of reduced blood volume (due to dehydration) and increased blood displacement to cutaneous vascular beds (due to heat stress) is decreased central venous pressure, venous return and, ultimately, cardiac output below euhydration values.135,169
Environmental Heat Stress, Dehydration, and Performance
Physiologic factors that contribute to dehydration-mediated aerobic exercise performance decrements include increased body core temperature, increased cardiovascular strain, increased glycogen utilization, and perhaps altered central nervous system function.141,166,175 Although each factor is unique, evidence suggests that they interact to contribute in concert, rather than in isolation, to degrade aerobic exercise performance.35,166,175 The relative contribution of each factor may differ depending on the specific activity, environmental conditions, heat acclimatization status, and athlete prowess, but elevated hyperthermia probably acts to accentuate the performance decrement.
In a field or wilderness setting, individuals may perform activities that require anaerobic power or muscular strength. Dehydration likely does not degrade muscular strength58,77 or anaerobic performance.34,85 However a recent critical review of the related literature89 suggests that hypohydration decreases (1% to 3%) strength, power, and high-intensity endurance activities. Dehydration >2% of body mass has been shown to degrade aerobic exercise performance in temperate, warm, and hot environments.31,37 As the level of dehydration increases, aerobic exercise performance is degraded proportionally.84 The critical water deficit (>2% body mass for most individuals) and magnitude of performance decrement are likely related to environmental temperature, exercise task, and the individuals’ unique biologic characteristics (e.g., tolerance to dehydration). Therefore, some individuals are more or less tolerant to dehydration. Adolph3 was one of the first to document that during long duration exercise in temperate or slightly warm environments, thermoregulatory sweating would lead to progressive dehydration and result in lower exercise output (Figure 70-5). Adolph derived this figure from limited exercise capability data and heart rate responses from a variety of exercise, heat stress, and dehydration conditions.

FIGURE 70-5 Percentage decrement in exercise performance relative to percent dehydration (body mass loss).
(Redrawn from Adolph EF: Physiology of man in the desert, New York, 1947, Interscience.)
Exercise tasks that primarily require aerobic metabolism and that are prolonged will be more adversely influenced by dehydration than are exercise tasks that require anaerobic metabolism or muscular strength and power.171 As demonstrated by Gonzalez-Alonso and colleagues,72 the greater the level of dehydration, the greater the magnitude of cardiovascular and thermoregulatory strain. It has previously been demonstrated that high levels of aerobic fitness and acclimatization status provide some thermoregulatory advantage. However, dehydration seems to cancel out this protective effect during exercise heat stress.25,119,172 A comprehensive review164 of a number of studies that investigated the impact of dehydration on physical exercise capacity and maximal aerobic power found that in the majority of studies, exercise capacity decreased with levels of dehydration of as little as 1% to 2% body mass, although maximal aerobic power was not altered. In addition, the reduction in exercise capacity when dehydrated was further accentuated by combination with heat stress.51,140,150 In temperate environments, body water deficits of <2% body mass did not have a significant impact on maximal aerobic power. In a hot environment, however, a small to moderate water deficit (≥2% body mass) resulted in a large decline in maximal aerobic power.51,140 A review of studies37 that observed the effects of progressive dehydration (>2% body mass) specifically on aerobic exercise performance found that in environments of >30° C (86° F), aerobic exercise performance was decreased by anywhere from 7% to 60%. It also appears that the magnitude of the effect increases as exercise extends beyond 90 minutes. Overall what can be taken from this review is that the impact of dehydration on prolonged work efforts is magnified by hot environments, and probably worsens as the level of dehydration increases.
Few investigations have studied the impact of dehydration on aerobic performance across a range of environmental temperatures. Cheuvront and co-workers25 observed 8% reduction in total work during a cycling time trial when dehydrated by ≥2% of body mass in a 20° C (68° F) environment. However, in 2° C (35.6° F), no effect of dehydration was observed. Kenefick and associates92 reported decrements in aerobic performance (15-minute cycling time trial) of −3%, −5%, −12%, and −23% in 10° C (50° F), 20° C (68° F), 30° C (86° F), and 40° C (104° F) respectively, when volunteers were dehydrated by ≥2% of body mass. Figure 70-6 depicts the change in performance relative to euhydrated trials and relative to the coefficient of variation (test variability; shaded area) of the cycling test itself. Mean values that lie inside of the shaded area are considered to be within the noise of the test and those that lie outside are considered to be meaningful. Based on the findings, it would appear that the temperature cusp where dehydration of 4% of body mass altered aerobic exercise performance occurred at 20° C (68° F). It is important to note that these reported results are the minimal decrement in performance that could be expected. Greater decrements could be expected during more prolonged work or with greater levels of dehydration.
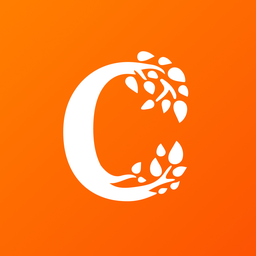
Full access? Get Clinical Tree
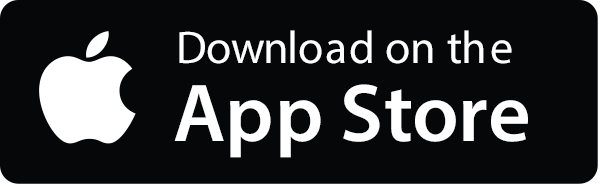
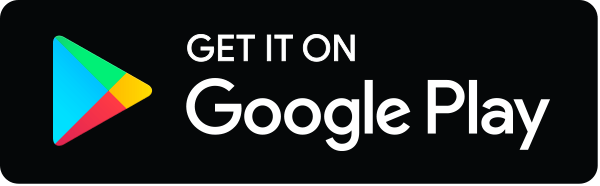