Chapter 38 Control of Breathing and Acute Respiratory Failure
Normal Regulation of Breathing
Rhythmic discharges whose timing corresponds to inspiratory and expiratory phases are generated in motor neurons of the medulla oblongata.1 During comfortable unstimulated breathing, inspiratory motor activity includes diaphragm flattening as well as a mild increase in pharyngeal muscle tone and vocal cord abduction that keep the upper airway patent during inspiratory airflow. When inspiratory muscles relax, resting expiration is passive, driven by elastic recoil of the lung and chest wall. The respiratory rate and motor intensity are modified by a variety of neural, chemical, and mechanical stimuli (Figure 38-1). With high-intensity stimulation, accessory muscles are activated, including intercostals and neck muscles. Nasal flaring occurs. Stimulated breathing also may include end-inspiratory vocal cord closure that acts to prolong lung inflation with little energy expenditure, clinically evident as grunting when the vocal cords open at the beginning of expiration. In highly stimulated breathing, abdominal muscles contract to force expiratory airflow.
Hypoxemia is a powerful stimulus to ventilation mediated by sensory input originating in the carotid body chemoreceptor. Peripheral chemoreceptor activity and ventilation increase slightly as Pao2 falls below 500 mm Hg. Ventilation rises steeply as Pao2 falls below 50 mm Hg (Figure 38-2, A). Low oxygen tension, rather than low oxygen, content is the ventilatory stimulus. Little carotid body response results from profound anemia. Hydrogen ion concentration and carbon dioxide tension independently activate chemoreceptors in the carotid body and in the brainstem (Figure 38-2, B). The simultaneous presence of hypoxia augments the hypercapnic ventilatory response (Figure 38-2, C).
Sleep modifies breathing, and compensation for respiratory illness is most likely to fail during sleep.2 In some persons ventilatory responses to hypercapnia and hypoxia diminish during sleep. Sleep-induced reduction in upper airway tone and cough reflexes worsens the risk of obstruction and aspiration. In infants, whose thorax is compliant, awake lung volume is maintained by thoracic muscle tone and breathing at sufficiently high frequencies that expiration seldom reaches the passive resting lung volume. During sleep, inspiratory muscle tone diminishes and respiratory rate decreases, with resulting reduction in infants’ expiratory lung volume. Infants’ compensation for mechanical loads is compromised during the rapid eye movement stage of sleep more than during quiet sleep. Although sleep is a period of high risk for the sick infant, depriving the patient of sleep is counterproductive. Obstructive and central apnea are worsened by sleep deprivation in healthy infants.3
Genetic factors may account for some variation in respiratory regulation in a normal population,4 but the clinical importance of this variation in predisposing individuals to acute respiratory failure is not clear. Although apnea is common in the premature infant, immaturity of respiratory controls does not otherwise appear to be a risk factor for respiratory failure in infant populations.
Failure of Respiratory Controls
Acute Disorders of Respiratory Controls
Patients with a critical illness or injury generally hyperventilate. At least some of the increased respiratory drive can be attributed to a higher metabolic rate. Pain, discomfort, and fear also stimulate ventilation. When a stressed patient fails to hyperventilate, depressed respiratory controls and impending respiratory failure should be suspected.
Moderate brain injuries typically are associated with hyperventilation (Figure 38-3, B), whether the injury is traumatic, infectious, or hypoxic-ischemic. The hypermetabolic state, lung pathology, and loss of inhibitory cortical influences probably combine to augment ventilation. Even when the brain-injured patient does hyperventilate, airway protective reflexes usually are impaired, seizures may ensue, and subtle progression of the brain lesion may lead to hypoventilation (Figure 38-3, A, CtoE). Resulting hypoxia may exacerbate the brain injury.
Respiratory depression by analgesic drugs, sedative agents, anticonvulsant medications, and anesthetic drugs is common. Opiates, benzodiazepines, barbiturates, and propofol all have respiratory-depressing effects. Relative effects on upper airway patency and hypoxic, hypercapnic, and loading responses may be dissociated. For example, Chloral hydrate has little effect on chemosensitivity but reduces genioglossus muscle tone and predisposes to obstructive apnea. Concern regarding respiratory depression does not warrant withholding analgesia. Rather, monitoring should be appropriate. In fact, episodic hypoxia during treatment procedures may be reduced when appropriate analgesia is provided.5 Sedative agents and analgesic drugs that are rapidly cleared after a single dose may have a more prolonged duration of action when given repeatedly or continuously. Clearance rates for medications may vary with systemic disease, immaturity, or genetic factors. Sedation and analgesic-induced respiratory depression may prolong the need for mechanical ventilation. Substituting an agent that clears rapidly (such as remifentanil) for longer acting agents several hours before a planned extubation may facilitate weaning from mechanical ventilation. Dexmedetomidine, an α2 adrenergic agonist, may provide sedation with less respiratory depression than other agents.
Other medications may depress breathing without alteration of consciousness. For example, prostaglandin E1, which is given to maintain patency of the ductus arteriosus in infants with congenital heart disease, is frequently associated with respiratory depression.6
As the severely dyspneic patient decompensates, exhausted efforts may rapidly give way to periodic breathing and apnea. While this phenomenon is commonly observed in infants with lower respiratory infections7 and pertussis,8 observations in adults with near-fatal asthma reveal a similar tendency for respiratory arrest to precede cardiovascular collapse.9 The mechanism of this preterminal respiratory depression is not well understood, but it appears to occur in some patients prior to the development of hypoxia and hypercapnia.
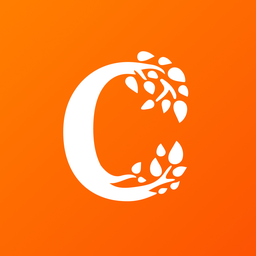
Full access? Get Clinical Tree
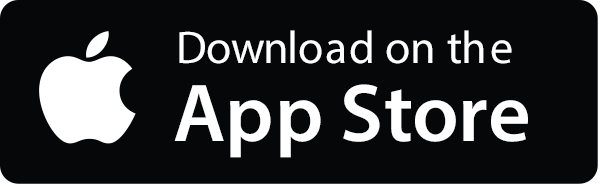
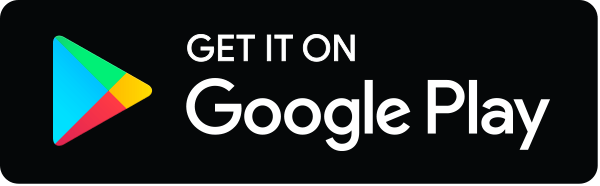