Figure 6-1. Skulls showing knife marks from ritual trepanning. This procedure was intended to release spirits causing headaches and other cranial ailments. (Photograph © Rosamond Purcell. Originally published in Mütter Museum calendar, College of Physicians, Philadelphia, PA, 1993.)
By the sixteenth century, the conquistadors had disrupted Incan society and began paying laborers with cocaine paste. The laborers generally rolled the cocaine leaves into balls (called cocadas), bound together by guano or cornstarch.1,2 These cocadas released the free-base cocaine as a consequence of the alkalinity of the guano and of the practice of chewing the cocadas with ash or lime (such alkaline compounds increase pH, favoring the free-base cocaine form over the positively charged hydrochloride salt). This practice probably marks the birth of “free-basing” cocaine and is the historic antecedent of the “rock” or “crack” cocaine so often abused in Western societies.
Cocaine was brought back to Vienna by an explorer/physician named Scherzer.1 In Vienna, the chemist Albert Niemann isolated and crystallized the pure cocaine hydrochloride in 1860. The Merck Company distributed batches of this agent to physicians for investigational purposes. Sigmund Freud was the most prominent of these cocaine experimenters. Freud reviewed his experimental work in a monograph devoted to cocaine titled Über Coca. Freud and Carl Koller (an ophthalmology trainee) took cocaine orally and noticed that the drug rendered their tongues insensible. Koller and Joseph Gartner began a series of experiments using cocaine to produce topical anesthesia of the conjunctiva. The birth of local and regional anesthesia dates from 1884 when Koller and Gartner reported their success at producing topical cocaine anesthesia of the eye in the frog, rabbit, dog, and human.2–4
The use of local anesthesia quickly spread around the world. The American surgeon William Halsted at Roosevelt Hospital in New York reported using cocaine to produce mandibular nerve block in 1884 and to produce brachial plexus block less than a year later.5 These blocks were accomplished by surgically exposing the nerves, then injecting them under direct vision. Leonard Corning injected cocaine near the spine of dogs, producing what was likely the first epidural in 1885. Spinal anesthesia was first accomplished in 1898 by August Bier. Caudal epidural anesthesia was introduced in 1902 by Sicard and Cathelin.5 Cocaine spinal anesthesia was used to treat cancer pain in 1898. Bier described intravenous regional anesthesia in 1909. In 1911, Hirschel reported the first three percutaneous brachial plexus anesthesias. Fidel Pages reported using epidural anesthesia for abdominal surgery in 1921.
Cocaine was incorporated into many other products, including the original formulation of Coca-Cola devised by Pemberton in 1886. Wine tonics and other “patent” medicines of the day commonly contained cocaine (Figure 6-3), until the early 1990s when governments began regulating the use of cocaine.
MEDICINAL CHEMISTRY
All local anesthetics (LAs) contain an aromatic ring and an amine at either end of the molecule, separated by a hydrocarbon chain, and either an ester or an amide bond (Figure 6-4).3,4,6,7 Cocaine is the archetypical ester LA and is the only naturally occurring one. Procaine, the first synthetic ester LA, was introduced by Einhorn in 1904 (Table 6-1) 2 The introduction of the amide LA lidocaine in 1948 was transformative. Lidocaine quickly became used for all forms of regional anesthesia. Other amide LAs based on the lidocaine structure (prilocaine, etidocaine) subsequently appeared. A related series of amide LAs based on 2’, 6’-pipecoloxylidide was introduced (mepivacaine, bupivacaine, ropivacaine, and levobupivacaine). Ropivacaine and levobupivacaine are the only single-enantiomer (single optical isomer) LAs. Both are S(−)-enantiomers, which avoid the increased cardiac toxicity associated with racemic mixtures and the R(+)-isomers (this will be discussed in a subsequent section). All other LAs either exist as racemates or have no asymmetric carbons.
Figure 6-2. A ceremonial tumi knife of the same shape and size as the less ornate ones that were used by the Incas to bore holes through the skull (trepanning). (Reprinted, with permission, from Sabbatini RME: Brain & Mind Magazine, 1997, June.)
Figure 6-3. Examples of products that incorporated cocaine during the time before it became a controlled substance. Wines fortified with cocaine were particularly popular as “tonics.” (Reprinted, with permission, from Addiction Research Unit, University of Buffalo.)
Clinical Pearls
All LAs contain an aromatic ring and an amine at either end of the molecule, separated by a hydrocarbon chain, and either an ester or an amide bond.
BIOPHYSICS OF VOLTAGE-GATED Na CHANNELS & LAs
Studies of the mechanisms of LA action on nerves are studies of interactions between LAs and voltage-gated Na channels, since Na channels contain the LA binding site. Na channels are integral membrane proteins that initiate and propagate action potentials in axons, dendrites, and muscle tissue; initiate and maintain membrane potential oscillations in specialized heart and brain cells; and shape and filter synaptic inputs. Na channels share structural features with other voltagegated ion channels in their genetic “superfamily,” wich includes voltage-gated Ca and K channels. Na channels contain one larger α-subunit and one or two smaller β-subunits, depending on the species and the tissue of origin. The α-subunit, the site of ion conduction and LA binding, has four homologous domains each with six α-helical membrane- spanning segments (Figure 6–5).8,9 The external surface of the α-subunit is heavily glycosylated, which serves to orient the channel properly within the plasma membrane (Figure 6-6).
Figure 6-4. Structures of commonly used local anesthetics.
Chronology of Amide Local Anesthetic Development
Agent | Initial Investigator | Date Introduced |
| ||
Cocaine | Niemann | 1860 |
| ||
Benzocaine | Salkowski | 1895 |
| ||
Procaine | Einhorn | 1904 |
| ||
Dibucaine | Meischer | 1925 |
| ||
Tetracaine | Eisler | 1928 |
| ||
Lidocaine | Lofgren, Lundquist | 1943 |
| ||
Chloroprocaine | Marks, Rubin | 1949 |
| ||
Mepivacaine | Ekenstam | 1956 |
| ||
Bupivacaine | Ekenstam | 1957 |
| ||
Etidocaine | Adams, Kronberg, Takman | 1972 |
| ||
Ropivacaine | Ekenstam, Sandberg | 1996 |
| ||
Levobupivacaine | Ekenstam and others | 1999 |
Invertebrates have only one or two Na channel α-subunit genes. Humans, in contrast, have nine active Na channel α-subunit genes on four chromosomes, with cell-specific expression and localization of gene products. The Nav 1.4 gene (by convention, geneticists refer to voltage-gated Na-channel isoforms as Nav 1 .x) supplies channels to skeletal muscle, and the Nav 1.5 gene supplies channels to cardiac muscle, leaving seven Nav isoforms in neural tissue (Table 6-2). Defined genes contribute specific Na channel forms to each of unmyelinated axons, nodes of Ranvier in motor axons, and small dorsal root ganglion nociceptors.10 Whereas all Na channel α-subunits will bind local anesthetics similarly, not all of them will bind neurotoxins with the same affinity.
Figure 6-5. Structure of Na channel subunits. Note that the α-subunit has four domains that each contain six membrane-spanning segments. (Reprinted, with permission, from Plummer NW, Meisler MH: Evolution and diversity of mammalian sodium channel genes. Genomics 1999;57:323–331.)
Figure 6-6. Cartoon of a Na channel in the plasma membrane. Note that all three subunits are heavily glycosylated. In contrast to local anesthetics, note that both scorpion toxins (ScTX) and tetrodotoxin (TTX) have binding sites on the external surface of the channel. Note also that the cytoplasmic side of the channel is phosphorylated. (Reprinted, with permission, from Catterall WA: Cellular and molecular biology of voltage-gated sodium channels. Physiol Rev 1992;72(Suppl):515–548.)
Na channel α- and β-subunit mutations lead to muscle, cardiac, and neural diseases.11 For example, inherited mutations in Nav 1.5 have been associated with congenital long QT syndrome, Bruguda syndrome, and other conduction system diseases.11 It has been shown that certain Nav isoforms proliferate in animal models of chronic pain. The existence of specific Nav gene α-subunit products offers the enticing possibility that inhibitors may some day be developed for each specific Nav α-subunit form. Such developments could revolutionize the treatment of chronic painful conditions.
CNS = central nervous system; DRG = dorsal root gamglion; TTX = tetrodotoxin.
Adapted, with permission, from Novakovic SD, et al. Regulation of Na+ channel distribution in the nervous system. Trends Neurosci 2001;24:473–478) (Table 1, page 474).
Blocking of impulses in a nerve fiber requires that a defined length of nerve become nonexcitable (to prevent the impulse from “jumping over” the blocked segment). Thus, as the LA concentration increases, it must need inhibit a shorter length of nerve to prevent impulse conduction, as is shown in Figure 6-7. Both normal conduction and LA inhibition of conduction differs between myelinated and unmyelinated nerve fibers. Conduction in myelinated fibers proceeds in jumps from one Ranvier node to the next, a process termed saltatory conduction. To block impulses in myelinated nerve fibers, it is generally necessary for LAs to inhibit channels in three successive Ranvier nodes (Figure 6-8). Unmyelinated fibers, lacking the saltatory mechanism, conduct much more slowly than myelinated fibers. Unmyelinated fibers are relatively resistant to local anesthesia, despite their smaller diameter, due to dispersal of Na channels throughout their plasma membranes. These differences among nerve fibers arise during development when Na channels begin to cluster at Ranvier nodes in myelinated axons. Nodal clustering of channels, essential for high-speed signal transmission, is initiated by Schwann cells in the peripheral nervous system and by oligodendrocytes in the central nervous system.12 It is the loss of clustering of Na channels in axons that underlies the electrophysiologic consequences of multiple sclerosis.
Figure 6-7. Note that the concentration of local anesthetic required to produce nerve block declines as the length of nerve exposed to the local anesthetic increases. (Reprinted, with permission, from Raymond SA, Steffensen SC, Gugino LD, et al: The role of length of nerve exposed to local anesthetics in impulse blocking action. Anesth Analg 1989;68:563–570.)
Na channels exists in at least three native conformations: “resting,” “open,” and “inactivated,” first described by Hodgkin and Huxley.13 During an action potential, neuronal Na channels open briefly, allowing extracellular Na ions to flow into the cell, depolarizing the plasma membrane. After only a few milliseconds, Na channels inactivate (whereupon the Na current ceases). Na channels return to the resting conformation with membrane repolarization. The process by which channels change from conducting to nonconducting forms is termed gating. Gating is assumed to result from movements of dipoles in response to changes in potential. The process by which voltage-gated channels operate likely involves movements of paddle-shaped voltage sensors within the channel’s outer perimeter (Figure 6-9).14,15 The speed of gating processes differs among Nav α-subunit forms: skeletal muscle and nerve forms gate quicker than cardiac forms.
Local anesthesia results when LAs bind Na channels and inhibit the Na permeability that underlies action potentials.6,13 Our understanding of LA mechanisms has been refined by several key observations. Taylor confirmed that LAs selectively inhibit Na channels in nerves under voltage clamp.16 Strichartz first observed use-dependent block with LAs, showing the importance of channel opening for LA binding. Use-dependence describes how LA inhibition of Na currents increases with repetitive depolarizations. Repetitive trains of depolarizations increase the likelihood that an LA will encounter an Na channel that is open or inactivated, both of which forms have greater LA affinity than do resting channels (Figure 6-10).6,13,17 Thus, membrane potential influences both Na channel conformation and Na channel affinity for LAs. Use-dependent block appears important for LAs functioning as antiarrhythmics and may also underlie the effectiveness of reduced LA concentrations in managing pain. Finally, using site-directed mutagenesis, Ragsdale and Wang have localized LA binding to specific amino acids in D4S6 of Nav 1.2 and Nav 1.4.18,19
Figure 6-8. Electron micrograph of a node of Ranvier. Na channels have been immunolabeled and appear as dense granules between the four arrows. The paranodal region is indicated by pn,” and an astrocyte is indicated by as.” (Reprinted, with permission, from Black JA, Friedman B, Waxman SG, et al: Immunoultrastructural localization of sodium channels at nodes of Ranvier and perinodal astrocytes in rat optic nerve. Proc R Soc Lond B Biol Sei 1989;238:39–51.)
Figure 6-9. In the conventional model for voltage-gating the voltage-sensing part of the channel slides in and out” of the membrane. More recent x-ray diffraction studies of the channel suggest that a more appropriate mechanism is that of paddle-like structures sliding diagonally through the plasma membrane. (Reprinted, with permission, from Århem P: Voltage sensing in ion channels: A 50-year-old mystery resolved? Lancet 2004;363:1221.)
Figure 6-10. Use-dependent block of Na currents in Purkinje fibers. Under control conditions each of a train of impulses results in identical currents. In the presence of the local anesthetic QX222, the first impulse is nearly the same size as under control conditions. Each succeeding impulse is smaller (reduced peak lNa), reflecting accumulating block of Na channels, until a nadir is reached. (Reprinted, with permission, from Hanck DA, Makielski JC, Sheets MF: Kinetic effects of quaternary lidocaine block of cardiac sodium channels: A gating current study. J Gen Physiol 1994;103:19–43.)
Figure 6-11. Reduced potency of S(-)-bupivacaine relative to R(+)-bupivacaine at inhibiting cardiac Na currents under voltage clamp. After a standard conditioning” depolarization of varying lengths, the S(-)-isomer produces less reduction of l/lmax than the R(+)-isomer. (Reprinted, with permission, from Valenzuela C, Snyders DJ, Bennett PB, et al: Stereoselective block of cardiac sodium channels by bupivacaine in guinea pig ventricular myocytes. Circulation 1995;92:3014–3024.)
Some LA optical isomers confer greater apparent safety than their opposite enantiomer. For example, under voltage clamp, the R(+)-bupivacaine isomer more potently inhibits cardiac Na currents than the S(-)-bupivacaine (levobupivacaine) isomer (Figure 6-11).
Many other types of chemicals will also bind and inhibit Na channels, including general anesthetics, substance P inhibitors, α2-adrenergic agonists, tricyclic antidepressants, and nerve toxins.6,20–22 The latter two chemical classes have undergone animal and early human testing as possible replacements for LAs. Unfortunately, several of the tricyclic antidepressants have demonstrated toxic side effects.
LA PHARMACODYNAMICS
In clinical practice LAs are typically described by their potency, duration of action, speed of onset and tendency for differential sensory nerve block. These properties do not sort independently.
Potency & Duration
Nerve-blocking potency of LAs increases with increasing molecular weight and increasing lipid solubility.23,24 Larger, more lipophilic LAs permeate nerve membranes more readily and bind Na channels with greater affinity. For example, etidocaine and bupivacaine have greater lipid solubility and potency than lidocaine and mepivacaine, to which they are closely related chemically.
Clinical Pearls
Nerve-blocking potency of LAs increases with increasing molecular weight and increasing lipid solubility.
More lipid-soluble LAs are relatively water-insoluble, highly protein-bound in blood, less readily removed by the bloodstream from nerve membranes, and more slowly “washed out” from isolated nerves in vitro. Thus, increased lipid solubility associates with increased protein binding in blood, increased potency, and longer duration of action. Extent and duration of anesthesia can be correlated with LA content of nerves in animal experiments.25,26 In animals, blocks of greater depth and longer duration arise from smaller volumes of more concentrated LA, compared wth larger volumes of less concentrated LA.27
Characteristics of Local Anesthetics
Physical and Chemical Increasing lipid solubility Increased protein binding |
|
Pharmacologic and Toxicologic Increasing potency Prolonged onset time Prolonged duration of action Increasing tendency to produce severe cardiovascular toxicity |
In general, all tend to sort together |
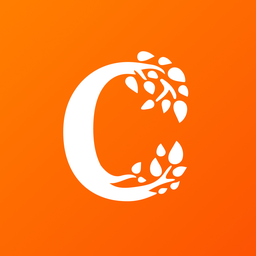
Full access? Get Clinical Tree
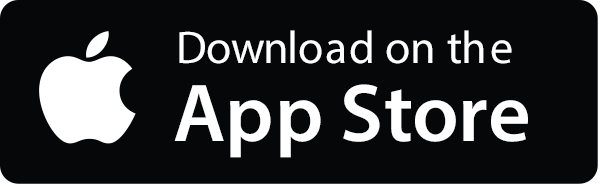
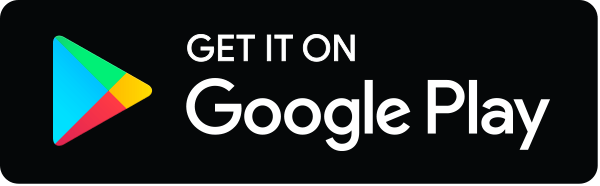