Abstract
High altitude has no consensus definition. It is most commonly defined as corresponding to >2500 m above sea level, where most individuals begin to show physiological adaptations. Long-term habitation at high altitude is possible – 100 million people live at altitudes >2500 m. Extreme altitude is defined as >6000 m – Mount Everest is 8848 m high. Humans are able to adapt their physiology to survive for short periods of time at extreme altitude, but long-term habitation is impossible.
High altitude has no consensus definition. It is most commonly defined as corresponding to >2500 m above sea level, where most individuals begin to show physiological adaptations. Long-term habitation at high altitude is possible – 100 million people live at altitudes >2500 m. Extreme altitude is defined as >6000 m – Mount Everest is 8848 m high. Humans are able to adapt their physiology to survive for short periods of time at extreme altitude, but long-term habitation is impossible.
What are the problems associated with high altitude?
High altitude presents a number of problems to the body, classified as:
Reduced barometric pressure (PB): during ascent, the PB decreases exponentially.
Temperature: the environmental temperature falls by 1°C for every 150 m elevation above sea level.
Reduced relative humidity: this results in increased evaporative losses from the skin and respiratory tract.
Increased solar radiation: due to reduced cloud cover.
How is alveolar oxygen tension PAO2 affected by altitude?
PAO2 is calculated using the alveolar gas equation (AGE) (see Chapter 18):

As the height above sea level increases, PB decreases exponentially, but the inspired fractional oxygen concentration remains the same (21%); PAO2 will also therefore decrease. In turn:
As alveolar gas is in equilibrium with the arterial gas, PaO2 will fall.
A fall in PaO2 results in lower haemoglobin (Hb) saturation (see Chapter 8), with the result that O2 carriage is reduced.
O2 delivery becomes insufficient for the tissues to maintain their normal metabolic processes; that is, hypoxaemic hypoxia develops.
Saturated vapour pressure of water is unchanged by altitude (6.3 kPa at 37°C), so it has a proportionally greater effect on PAO2 at higher altitudes, when PB is reduced.
How does the body adapt to survive at altitude?
Survival at altitude depends on a coordinated response by the respiratory, cardiovascular, haematological and renal systems. No physiological changes are seen below 2500 m in a healthy adult, probably because PaO2 is above the threshold for activation of the peripheral chemoreceptors (around 8 kPa).
The acute response to altitude produces a compensatory increase in PaO2 by hyperventilation, but its action is limited by the resulting respiratory alkalosis. This is followed by the chronic adaptive response, in which a further increase in ventilation is permitted as the kidney corrects the pH disturbance. System by system:
Respiratory system. Important aspects are:
Hyperventilation. The peripheral chemoreceptors (but not the central chemoreceptors) are sensitive to changes in PaO2 (see Chapter 22). When PaO2 falls below 8 kPa, the peripheral chemoreceptors stimulate the respiratory centre in the medulla, resulting in increased V̇A. The consequent fall in PaCO2 leads to an increase in PAO2, according to the AGE (see above).
‘Braking effect’. The unwanted consequence of hyperventilation is an increase in arterial pH; that is, a respiratory alkalosis. Hypocapnoea is detected by the central chemoreceptors, whilst alkalosis is detected by the carotid bodies; both act to limit the increase in V̇A.1
Diffusion limitation. Transfer of O2 across the alveolar–capillary barrier may become diffusion limited at altitude (see Chapter 10), especially in association with exercise (where the pulmonary capillary transit time is reduced) and in patients with high-altitude pulmonary oedema (interstitial fluid thickens the alveolar–capillary barrier).
Cardiovascular system. Key features are:
Increased heart rate (HR) as a result of increased sympathetic outflow triggered by the peripheral chemoreceptors.
Reduced plasma volume. Haematocrit increases due to a 20% reduction in plasma volume. This is the combined result of a pressure diuresis (an increase in renal perfusion driven by the increase in sympathetic activity), increased fluid loss (hyperventilation and reduced relative humidity) and decreased oral intake (loss of appetite). Stroke volume falls as a result of the reduction in preload, but overall cardiac output remains the same owing to tachycardia.
Increased myocardial work. The high haematocrit of the blood (up to 0.6 with chronic acclimatisation) increases the viscosity of the blood. The work required to move blood in the circulation (i.e. the left ventricular work) is increased.
Hypoxic pulmonary vasoconstriction (HPV). The pulmonary arterioles vasoconstrict in response to low PAO2 (see Chapter 23). Normally, HPV is a useful mechanism to optimise V̇/Q̇ matching when a region of the lung is hypoxic (e.g. due to a lobar pneumonia). However, the generalised hypoxia that occurs at altitude results in a potentially harmful global pulmonary arteriolar vasoconstriction. Pulmonary vascular resistance increases by 50–300%, resulting in pulmonary hypertension. The increase in pulmonary capillary hydrostatic pressure may cause fluid transudation, known as high-altitude pulmonary oedema (HAPE). In addition, the sudden onset of pulmonary hypertension may precipitate right heart failure in susceptible individuals.
Haematological system. The sigmoid shape of the oxyhaemoglobin dissociation curve is important: SaO2 remains >90% at elevations of up to 3000 m in normal patients. At higher altitudes, compensatory mechanisms occur:
Leftward shift of the oxyhaemoglobin dissociation curve. Hyperventilation-induced alkalosis shifts the P50 of the oxyhaemoglobin dissociation curve to the left. This aids the loading of scarce O2 onto the Hb molecule in the lungs, but prevents offloading of O2 to the tissues (see Chapter 8).
Increased 2,3-diphosphoglycerate (DPG). To compensate for the leftward shift of the oxyhaemoglobin dissociation curve, erythrocytes produce a greater amount of 2,3-DPG. This causes a rightward shift of the curve in order to facilitate O2 offloading from Hb, returning the P50 to the normal sea-level position within a week.
Increased red cell mass. The kidney responds to chronic hypoxaemia within hours of ascent by increasing the secretion of erythropoietin, which stimulates erythrocyte production by the bone marrow. Over time, red cell mass increases, restoring the blood’s O2-carrying capacity to near normal.
Increased risk of thrombotic events. This is as a result of both the increase in haematocrit (which increases the viscosity of the blood) and the activation of platelets due to hypoxaemia.
Thermoregulation. Continuous exposure to a cold environment causes a number of metabolic changes:
Heat conservation. Mechanisms include peripheral vasoconstriction, decreased sweating and behavioural changes (e.g. wearing more clothes).
Heat generation. Mechanisms include increasing basal metabolic rate, shivering and increased brown fat activity (in infants). All of the heat-generating mechanisms increase O2 consumption at a time of relative hypoxaemia.
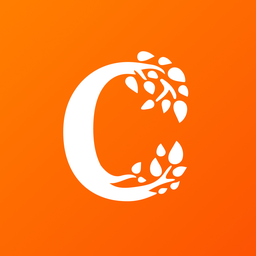
Full access? Get Clinical Tree
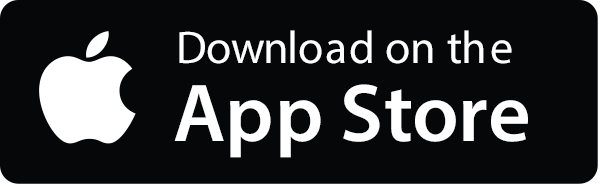
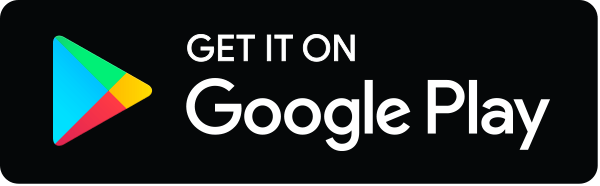