Abstract
Oxygenation is the primary aim of all airway management. The shape of the oxyhaemoglobin dissociation curve determines onset of hypoxaemia and means that when it occurs it can progress rapidly and severely. Hypoxaemia causes rapid damage to living tissues, most importantly the brain. Oxygenation strategies are required including delivering oxygen before, during and after airway management. Low flow oxygenation techniques are traditionally employed and apnoeic oxygenation has been rarely used. Newer high flow oxygen delivery systems enable effective pre-oxygenation and apnoeic oxygenation and can be used both during complex airway management or as an alternative to instrumenting the airway during surgery.
Introduction
Hypoxia is one of the most serious risks facing patients during anaesthesia, with prolonged hypoxia leading to arrhythmia, haemodynamic decompensation, hypoxic brain injury and death. Preventing hypoxia throughout airway management is a major priority for all clinicians who manage the airway. Patients can experience hypoxia during induction, maintenance and recovery from general anaesthesia. To counter the risk of hypoxia during airway management pre-oxygenation, which is an essential component of airway management, is recommended in all patients before induction of general anaesthesia, management of the airway or extubation.
In this chapter, we will review pre-oxygenation and peri-intubation oxygenation techniques, which may reduce the risk of critical hypoxia during airway management.
Pre-oxygenation
Pre-oxygenation is the administration of oxygen prior to induction of general anaesthesia and airway management and is considered a minimum standard of care. The main goal of pre-oxygenation is to delay the onset of oxyhaemoglobin desaturation during apnoea, often referred to as the ‘safe apnoea time’. The safe apnoea time can be defined as the period of time until critical arterial oxygen desaturation (SaO2 88–90%) occurs following cessation of breathing/ventilation. Arterial saturation at 88–90% marks the upper inflection point on the oxygen–haemoglobin dissociation curve beyond which further decreases in PaO2 lead to a rapid decline in SaO2 (≈30% every minute) (Figure 8.1).
During apnoea oxygen consumption is approximately 200–250 mL min−1 (≈3 mL kg−1 min−1). The physiological objective of pre-oxygenation is to increase the total body store of oxygen thereby increasing the time until depletion, and hypoxia, occurs. This is achieved by increasing the oxygen stores in the lungs, blood, tissue fluids and in combination with myoglobin. The most significant increase takes place in the lungs where replacing nitrogen in the functional residual capacity (FRC) with oxygen (often referred to as ‘denitrogenation’) can increase oxygen stores from ≈450 mL breathing air to 3000 mL breathing 100% oxygen.
The FRC is the most important oxygen store in the body and in simple terms the greater the FRC, the longer the safe apnoea time. In a healthy pre-oxygenated patient the safe apnoea time is up to 8 minutes, compared to ≈1 minute breathing air. There are multiple conditions that will decrease safe apnoea time. These include
conditions leading to reduced FRC (e.g. obesity, pregnancy, intra-abdominal pathology, ventilation/perfusion mismatching)
airway occlusion
increased oxygen consumption
anaemia
dyshaemoglobinaemia
inadequate pre-oxygenation.
Many of these conditions frequently coexist in critically ill patients
Efficacy and Efficiency
The efficacy of pre-oxygenation relates to the ability to achieve end-tidal oxygen (ETO2) > 90% and is dependent on the anaesthesia circuit used, oxygen flow rate, FRC and alveolar ventilation (V̇A).
The efficiency of pre-oxygenation is defined by the rate of decline in arterial oxygen saturation and ultimately this is what is important in pre-oxygenation, i.e. the extension of the safe apnoea time. This is dependent on (i) the efficacy of pre-oxygenation (oxygen stored at the beginning of apnoea), (ii) the capacity for additional oxygen to be bound to haemoglobin during apnoea (e.g. by apnoeic oxygenation) and (iii) oxygen consumption (which is increased in many conditions including sepsis, pyrexia, pregnancy, obesity and in infants).
As an example, it is useful to consider the efficacy and efficiency of pre-oxygenation in a pregnant mother. She has good efficacy with her increased alveolar ventilation (rapidly filling her lung) and reduced FRC (less lung to fill), but poor efficiency with rapid oxygen desaturation due to reduced FRC (reduced oxygen store) and increased oxygen consumption.
ETO2 monitoring is the gold standard in clinical practice for assessing denitrogenation of the lungs during pre-oxygenation and is a measure of efficacy. Optimal efficacy is achieved with ETO2 > 90%, although this may not be achieved in some patients regardless of the method or duration of pre-oxygenation.
Methods
Methods of pre-oxygenation can be divided into slow and fast techniques. The rate of denitrogenation depends on the inspired fraction of oxygen, the inspired tidal volume relative to the FRC, and the respiratory rate.
Slow technique: tidal-volume breathing technique. Provide 100% oxygen via a tight-fitting face mask and ask the patient to breathe at normal tidal volumes for three or more minutes. If there are difficulties in achieving a good seal, a two-handed mask technique can be applied to create a better seal. Pre-oxygenation may be augmented by asking the patient to fully exhale before pre-oxygenation starts.
Fast technique: deep breathing technique. Provide 100% oxygen via a tight-fitting face mask and ask the patient to take eight deep vital capacity breaths in 60 seconds. The alveolar oxygen fraction increases rapidly, but total tissue oxygenation may not be as great as with the slow technique. An important limitation to this technique is that in many anaesthetic circuits the vital capacity (up to 5 L) may far exceed the volume of the circuit reservoir bag (usually 2 L), which will completely empty during inspiration and continuing inspiratory efforts will cause atelectasis, so counteracting the benefits of pre-oxygenation. Using an extra-large reservoir bag, increasing oxygen flow or by deploying the oxygen flush throughout this period may counteract this limitation.
Devices
Oxygen delivery devices may be divided into low flow and high flow systems. The principal difference being that low flow oxygen systems depend on inspiration of room air to meet the patient’s inspiratory flow and volume demands, whereas high flow oxygen delivery systems are designed to provide the patient’s entire inspiratory demands by providing either large oxygen reservoirs or flow rates. Low flow devices include standard nasal cannula, simple face masks, partial rebreather masks, non-rebreather mask and tracheostomy collars (Figure 8.2). The FiO2 delivered by these systems depends on the degree of mixing of delivered oxygen with ambient air. This in turn depends on the patient’s ventilatory pattern (tidal volume, peak inspiratory flow, respiratory rate and minute ventilation), the size of any device oxygen reservoir and the oxygen flow rate. These devices therefore deliver an unpredictable FiO2 and are less suitable for optimal pre-oxygenation than high flow devices.
Low flow oxygen delivery systems may be used in combination (e.g. nasal cannula and non-rebreather mask) or at very high flow rates to provide efficient pre-oxygenation. Non-rebreather masks may thus provide an FiO2 ≥ 0.9 by increasing the oxygen flow rate to 30–60 L min−1 and provide a similar FiO2 as bag-valve-mask (BVM) delivering 15 L min−1. A BVM may be subjectively more difficult to breathe through and cause hypoventilation or rebreathing.
High flow oxygen delivery systems have flow rates and reservoirs large enough to provide the total inspired gases regardless of the patient’s respiratory pattern. High flow devices include anaesthesia circuits, manual resuscitation bags with one-way valves on the expiratory port, aerosol masks and T-pieces that are powered by air-entrainment nebulisers or air-oxygen blenders and Venturi masks (Figure 8.2). These devices provide a fixed FiO2 and most are capable of delivering an FiO2 of 1.0. The primary limitations in regard to pre-oxygenation are cost, availability and bulkiness.
Positioning
Patients should be pre-oxygenated sitting up whenever possible.
In spontaneously breathing patients, a fully supine position will cause the diaphragm to move cephalad leading to compression atelectasis of the lower and dependent parts of the lung. The resulting reduction in FRC of 0.5–1.0 L will increase ventilation–perfusion mismatch (leading to hypoxia) and reduce pulmonary compliance. With increasing body mass index (BMI) and in pregnancy FRC and lung compliance are reduced with an exponential decrease in the oxygenation index (PaO2/PAO2).
Elevating the head of the bed reduces atelectasis and several studies have shown that the time to desaturation is increased by 20–30% in both normal-weight and obese patients by positioning the patient with the head of the bed elevated or in reverse Trendelenburg position.
An additional benefit of head elevation may be better laryngeal exposure during direct laryngoscopy, improved ease of intubation and reduced odds of intubation-related complications (see Chapter 14).
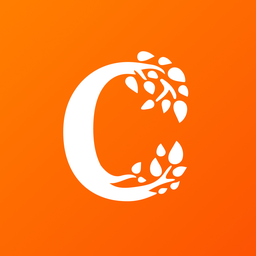
Full access? Get Clinical Tree
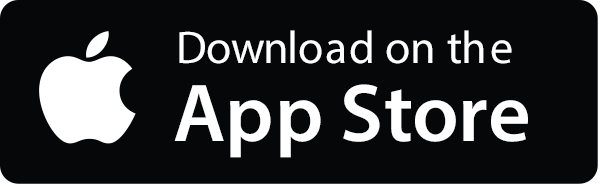
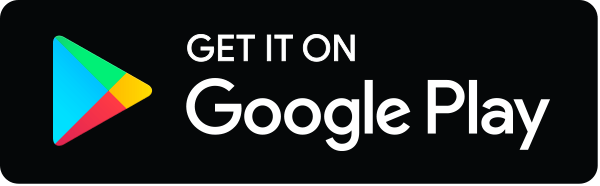