Abstract
Metabolism refers to the whole range of biochemical reactions that occur within living organisms. Metabolism broadly encompasses anabolism (the building up of larger molecules from smaller ones) and catabolism (their breaking down into smaller entities with the extraction of energy).
Metabolism refers to the whole range of biochemical reactions that occur within living organisms. Metabolism broadly encompasses anabolism (the building up of larger molecules from smaller ones) and catabolism (their breaking down into smaller entities with the extraction of energy).
What is meant by the term ‘cellular respiration’?
Cellular respiration is the series of catabolic processes by which carbohydrates, fats and proteins are broken down to yield ATP through a series of redox reactions, ultimately using O2 as the oxidising agent. As O2 is too reactive to be used directly, this process employs a series of intermediate electron carriers, including nicotinamide adenine dinucleotide (NAD+) and flavin adenine dinucleotide (FAD).
How are carbohydrates, fats and proteins metabolised to adenosine triphosphate?
Details of the metabolic processes involved are complex, but an overview remains useful for clinical practice. Catabolism involves a number of processes:
Glycolysis, the process by which glucose is converted to pyruvate, which then enters the citric acid cycle. Glycolysis takes place in the cytoplasm and can occur in either aerobic or anaerobic conditions.
Lipolysis, the process by which free fatty acids are oxidised to acetyl-CoA, which then enters the citric acid cycle.
Protein catabolism, a process of oxidative deamination in which amino acids have their amino groups removed to form keto acids. The keto acids may then enter the citric acid cycle or be converted to glucose or fatty acids. The amino groups are converted to urea by the urea cycle.
The citric acid cycle, in which acetyl-CoA and other metabolites are broken down through a series of redox reactions within the inner mitochondrial matrix. The resulting NADH, FADH2 and H+ are then processed by the electron transport chain.
The electron transport chain, located within the inner mitochondrial matrix, is the final step of aerobic metabolism. NADH and FADH2 transfer electrons to O2, releasing energy that is used to pump H+ across the inner mitochondrial membrane. The resulting electrochemical gradient is used to generate ATP.
Describe the important steps of the glycolytic pathway
Glycolysis (also called the Embden–Meyerhof pathway) is the metabolic pathway through which glucose is converted into pyruvate, with the generation of two ATP and two NADH molecules (Figure 77.1). Key features of the glycolysis pathway are:
Glycolysis occurs in the cytoplasm.
The first step is the phosphorylation of glucose, resulting in glucose-6-phosphate – the ‘active’ form of glucose. One ATP molecule is consumed and converted to ADP in this process. This reaction is catalysed by the enzymes glucokinase (in the liver) or hexokinase (in the other tissues).1 Phosphorylation of glucose maintains the low cytoplasmic concentration of glucose. This thereby maintains the concentration gradient for glucose diffusion from the extracellular to the intracellular space.
Glucose-6-phosphate (a six-carbon molecule) is broken down to two molecules of pyruvate (a three-carbon molecule) through a series of seven intermediates. During this process, a further molecule of ATP is consumed to form ADP, and then four molecules of ATP are produced from ADP.
The overall glycolysis reaction is
C6H12O6 + NAD+ + 2ADP + 2Pi → 2C3H4O3 + 2H+ + 2ATP + 2H2O + 2NADHC6H12O6+NAD++2ADP+2Pi→2C3H4O3+2H++2ATP+2H2O+2NADH
It is important to note that O2 is not consumed and CO2 is not produced. Glycolysis can therefore occur under both aerobic and anaerobic conditions.
NAD+ is required for glycolysis. This is where aerobic and anaerobic conditions differ:
– Under aerobic conditions: NADH produced during glycolysis exchanges electrons with NAD+ or FAD across the mitochondrial wall, which regenerates NAD+, thus allowing glycolysis to continue. Pyruvate then passes into the mitochondrion, where it enters the citric acid cycle.
– Under anaerobic conditions: the electron transport chain is not active, so there are no NAD+ or FAD molecules within the mitochondrion with which to exchange electrons. Glycolysis can only continue if NAD+ is regenerated through a different reaction: the production of lactic acid. Pyruvate is reduced to lactate by NADH in a reaction catalysed by the enzyme lactate dehydrogenase. This regenerates NAD+ in order that glycolysis can continue:
Pyruvate + NADH → Lactate + NAD+Pyruvate+NADH→Lactate+NAD+
Lactate has a number of fates:
▪ If the PO2 is restored, it can be oxidised back to pyruvate and enter the citric acid cycle.
▪ Lactate can leave the cell cytoplasm and travel in the circulation to the liver, where it can be either oxidised back to pyruvate or converted to glucose through a process called gluconeogenesis. This is known as the Cori cycle.
▪ In organisms without a liver (e.g. yeast), lactate is converted to ethanol to regenerate NAD+ in a process called fermentation.
An important intermediate in the glycolytic pathway is 1,3-bisphosphoglycerate (1,3-BPG). 1,3-BPG isomerises to 2,3-diphosphoglycerate (2,3-DPG), a molecule that binds strongly to deoxyhaemoglobin. Under conditions of low PO2, the rate of glycolysis becomes increased, as the enzyme phosphofructokinase involved in glycolysis is O2 sensitive. This results in increased 2,3-DPG levels, which aids the offloading of O2 from haemoglobin (Hb) (see Chapter 8).
Figure 77.1 The glycolytic pathway (simplified).
Lactic acidosis usually results from either regionally or globally reduced O2 delivery, such as in limb ischaemia, mesenteric ischaemia, cardiac arrest and severe shock (septic, cardiogenic, etc.). However, it is important to remember that lactic acidosis may occur for other reasons, including:
Reduced clearance of plasma lactate. This may occur in hepatic or renal dysfunction. Metformin (a biguanide) reduces hepatic gluconeogenesis. As lactate is used by the liver as a substrate for gluconeogenesis, metformin reduces hepatic lactate uptake, thus exacerbating a lactic acidosis.
Cytotoxic hypoxia. The electron transport chain can be poisoned by substances such as cyanide. Cells are then no longer able to utilise O2 for aerobic respiration and are reliant on glycolysis for ATP production.
Treatment of lactic acidosis is focused on diagnosis and correction of the underlying cause; this may involve surgery or organ support (by O2 administration, fluid or inotropic support, haemofiltration, etc.). Rarely, administration of sodium bicarbonate may be indicated if there is cardiovascular compromise as a result of profound acidosis or severe hyperkalaemia accompanying the acidosis.
What are the important steps of the citric acid cycle?
The citric acid cycle (also known as the Krebs cycle and the tricarboxylic acid cycle) takes place in the inner mitochondrial matrix. It involves a complex cycle of metabolic intermediates, producing CO2, ATP and electron donors (NADH and FADH2) that are then utilised in the electron transport chain (Figure 77.2). Whilst O2 is not consumed in the citric acid cycle, the cycle nevertheless ceases to operate under anaerobic conditions corresponding to a mitochondrial PO2 < 0.4 kPa. This is because the electron transport chain, which is dependent upon O2, is needed to regenerate NAD+ and FAD for use in the citric acid cycle.
The main substance consumed by the citric acid cycle is acetyl-CoA. Acetyl-CoA is a two-carbon molecule (the acetyl part) attached to the carrier coenzyme A (CoA or CoA-SH, derived from vitamin B). Acetyl-CoA is produced either from:
Pyruvate, according to the reaction:
Pyruvate (3) + CoA − SH + NAD+ → Acetyl − CoA (2) + CO2 + NADHPyruvate3+CoA−SH+NAD+→Acetyl−CoA2+CO2+NADH
(numbers in parentheses refer to number of carbon atoms in the molecule); or
β-oxidation of fatty acids – see below.
However, other substances (e.g. keto acids formed from the deamination of amino acids) can enter the citric acid cycle at different points.
The citric acid cycle is a complex system of eight molecular intermediates, enzymes and coenzymes. Key features of the citric acid cycle are:
Acetyl-CoA (2) reacts with oxaloacetate (4) to form citrate (6). Citrate is considered the starting point of the cycle (hence the name) and is traditionally drawn at the 12 o’clock position.
Citrate (6) (through an intermediate) decarboxylates to give α-ketoglutarate (5), NADH and CO2.
α-ketoglutarate (5) decarboxylates when it reacts with CoA to give succinyl-CoA (4), NADH and CO2.
The four-carbon succinyl-CoA undergoes a series of reactions and isomerisations, passing through a number of intermediate states before regenerating oxaloacetate (4). No CO2 is produced during these changes – the carbon number of the molecules is unchanged. However, the process does produce a molecule each of ATP, NADH and FADH2.
The NADH, FADH2 and H+ produced in the citric acid cycle are used in the electron transport chain to produce ATP.
The overall reaction for each acetyl group entering the citric acid cycle is:
Acetyl − CoA + 3NAD+ + FAD + ADP + Pi + 2H2O → CoA − SH + 3NADH + FADH2 + 3H+ + ATP + 2CO2Acetyl−CoA+3NAD++FAD+ADP+Pi+2H2O→CoA−SH+3NADH+FADH2+3H++ATP+2CO2
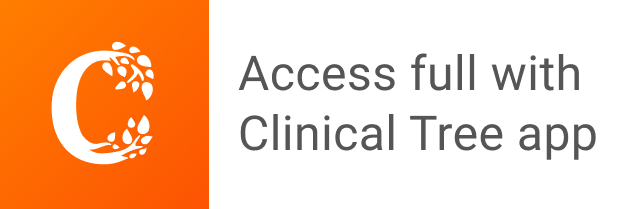