Introduction
As detailed within this book, perioperative neurocognitive disorder (PND) is a poorly understood complication of surgery and/or anesthesia that results in significant morbidity in the elderly, being associated with increases in hospital length of stay, decreases in quality of life, and an increase in mortality after surgery. Our lack of understanding of the mechanism and the neural correlates of POCD has hampered our ability to measure the impact on cognitive function, monitor its natural time course, and determine the effects of potential treatments.
Historically, neuroimaging studies in PND have focused on the development of new cerebrovascular white matter disease as a possible causal factor, but most investigations have shown significance only at a population level and have largely failed for individual subjects. Recently, efforts have shifted from strict anatomic imaging to a focus on identifying brain areas with altered function in the perioperative time frame. As described in other chapters, the links between PND and Alzheimer’s disease (AD) or neuroinflammation are particularly intriguing. Because of the relatively rich literature in the use of neuroimaging to diagnose and monitor cognitive dysfunction in these entities, this chapter will first describe the neuroimaging techniques used in AD and neuroinflammation by detailing the current state of the art in those fields to advance the idea that neuroimaging can be used to provide new insight into PND. We will then explore what has been published in regards to PND neuroimaging and how this may be expanded on in the future.
Imaging Basics
To appreciate how neuroimaging may be applied to the study of PND, the basic principles of several neuroimaging techniques should be briefly explained. Although arguments could be made for the role of computed tomography (CT), cranial ultrasound, near infrared spectroscopy, or magnetoencephalography, the two technologies that researchers have focused on are magnetic resonance imaging (MRI), and positron emission tomography (PET).
Magnetic Resonance Imaging
MRI utilizes magnetic fields and radio waves, and therefore is considered a noninvasive technology. As such, it would be well suited for the longitudinal studies necessary for PND investigation. MRI exploits the fact that materials are able to absorb and then emit radio frequency energy pulses when placed in a magnetic field. The emission of these waves is called relaxation, and the various types of tissue in the human body have different relaxation rates. By varying the magnetic field and the timing of the radio pulses, these relaxation rate differences can be used to generate two- or three-dimensional images that can highlight specific tissues, such as white matter, gray matter, cerebrospinal fluid (CSF), or blood, as well as processes such as blood flow and water diffusion.
MRI is able to generate high-resolution images of the brain in only a few minutes, with entire brain volumes of lower resolution images able to be acquired as quickly as 1s. When these lower resolution brain volumes are collected repeatedly, the technique is referred to as functional MRI (FMRI). A typical FMRI study focuses on the time course of alterations in the MRI signal caused by fluctuations in the magnetic susceptibility of deoxygenated blood, termed blood oxygen level-dependent (BOLD) contrast. Changes in the BOLD signal time course can be induced by task-related activity, either in a repeating-block design or an event-related fashion (1). Figure 13.1 displays results typical of a BOLD FMRI study, in this case the perception of a painful transcutaneous nerve stimulation (2).
Figure 13.1 Example of brain activation and BOLD signal time course for a 30 s on–30 s off pain task, showing activation in the primary and secondary somatosensory cortices (SI and SII). The activation is colored by z-score, in this case, from 3 to 8. One can see the MRI signal increase in the active areas when there is pain (indicated by the solid black line on the x-axis). The average signal time course is also displayed, showing the relative stability of the BOLD response.
Recently, a technique termed functional connectivity MRI (fcMRI) has become popular. fcMRI relies on spontaneous, low-frequency (<0.1 Hz) fluctuations in the BOLD signal over time. Although this approach has been used in a task-related fashion, its growth can be directly attributed to its use in “resting-state” imaging where these fluctuations reveal coherent networks of anatomically distinct yet functionally connected brain regions, even in the absence of experimental stimuli (3, 4). Resting-state fcMRI use as a biomarker in diseases such as depression (5), as shown in Figure 13.2, and Alzheimer’s disease (AD) (6) is growing as well.
Figure 13.2 Functional connectivity MRI (fcMRI) is based on the idea shown in the upper panel. A seed voxel is chosen, and the signal time course is extracted. That time course is then regressed against the rest of the brain, and areas with similar time course fluctuations are said to be “functionally connected.” The lower panel shows application of fcMRI in depression, identifying areas where patients with varied types of depression collectively differed from healthy controls. SS1 = primary somatosensory cortex. VLPFC = ventrolateral prefrontal cortex. OFC = orbitofrontal cortex. M1 = primary motor cortex. PPC = posterior parietal cortex. GP = globus pallidus. Thal = thalamus. VMPFC = ventromedial prefrontal cortex. sgACC = subgenual anterior cingulate cortex. Amyg = amygdala. vHC = ventral hippocampus. PHC = parahippocampal cortex. vStr = ventral striatum.
Positron Emission Tomography
In PET imaging, subjects are injected with a radioisotope. Perhaps the most common example is fluorine-18 fluorodeoxyglucose (FDG), a tracer that is taken up by cells using glucose and thereby used to identify areas with increased metabolism. As the tracer decays, it emits two gamma photons that can be detected by the PET scanner. The temporal and spatial detection of these photons is not exact, however, thus the spatial resolution of PET is poor compared to MRI or even CT. Because of this, PET may seem to be disadvantaged when compared to FMRI approaches. However, with the ability to incorporate radioactive isotopes of elements such as carbon or oxygen into many molecules, the tracer can be tailored to specific physiologic phenomenon to be investigated.
Compounds used for AD imaging include Pittsburgh Compound B (PiB) and florbetapir. PiB contains a carbon-11 atom and florbetapir contains the 18F atom; both localize to areas with significant amounts of amyloid-beta (Aβ) in the cerebral cortex (7). Often this form of imaging is combined with FDG-PET (8) and MRI in clinical practice for the diagnosing of a patient’s cause of dementia. New C-11 based compounds are currently being investigated that target the 18-kDa translocator protein (TSPO) as a marker for microglial activation, indicating areas of neuroinflammation (9).
Neuroimaging in Alzheimer’s Disease
Because both PND and AD are characterized by decreases in memory and executive function, parallels between the two have been sought for some time. Although the entirety of the literature is mixed, two recent studies with more than 160,000 patients support a relationship between anesthesia/surgery and AD (10,11). Furthermore, biochemical biomarkers associated with AD, namely, Aβ and tau, are altered in the perioperative period (with increases seen in both, as reviewed by Berger et al. (12)) and it has been suggested that low CSF Aβ may be a significant predictor of PND (13). Given the similarities and potential link between PND and AD, the use of AD-related neuroimaging techniques may provide important information in PND. Thus, the key neuroimaging modalities and findings in AD are presented below to support the hypothesis that neuroimaging research can also be important in PND.
Aβ detection has been the cornerstone of AD imaging. PiB shows increased retention in the frontal cortex, parietal cortex, lateral temporal cortex, posterior cingulate cortex/precuneus, thalamus, and striatum of those with AD when compared to healthy controls, as shown in Figure 13.3 (14–17). The sensitivity for AD of increased PiB has been shown to be greater than 98%; however, the specificity is only in the mid-60% range (18). Nonetheless, this high sensitivity has been useful in clinical research studies; those found to be “PiB negative” are typically used as age-matched controls as the presence of preclinical AD is considered “ruled out” (19).
Figure 13.3 Example of PiB distribution in a healthy control and in a patient with AD. Standardized uptake values (SUV) show high PIB retention in the frontal and temporoparietal cortices.
While other PET-based markers of Aβ have been developed that show increased specificity in terms of AD (reviewed in Saidlitz et al. (20)), the presence of Aβ is not sufficient for the diagnosis of AD, and treatments aimed at reducing Aβ in those with dementia have not shown the therapeutic benefit hoped for, perhaps because irreversible neurodegenerative damage has already occurred. One approach being tested is the use of PET markers of tau proteins, as their deposition is another major abnormality in AD pathology. In particular, investigators have used [18F]-AV-1451 (flortaucipir) binding in the hippocampus and other AD-related regions to distinguish patients with AD from healthy controls, and to show that Aβ interacts with tau to affect neurodegeneration (21). Flortaucipir has also been used to detect tau pathology in functional resting-state networks, showing that they overlapped networks previously reported to be altered in AD (22). Flortaucipir is not the only tau tracer, however, as work with [18F]-THK5351 has also shown promise, with levels reflecting AD symptoms and severity (23), although it is adversely affected by monoamine oxidase type B enzyme levels.
In the search for improved biomarkers, hippocampal volume has emerged as a strong candidate. In particular, atrophy of the hippocampus and entorhinal cortex is of particular value as a biomarker due to their involvement in the earliest stage of disease and strong correlation with neurofibrillary tangle pathology (24–27). Hippocampal volume is reduced in patients with mild cognitive impairment (MCI) by 10%–15% while those with mild and moderate AD show reductions of 15%–30% and 30%–40%, respectively (28,29). The rate of atrophy is also predictive of progression, being 4%–6% per year in MCI/AD patients compared to 1%–2% in age-matched controls (14).
If hippocampal volume change shows promise, can hippocampal function be used to help clarify the diagnosis? A recent study stratifying patients with MCI based on Aβ presence demonstrated that patients with positive Aβ scanning exhibited a higher level of hippocampal functional activity in response to a memory task and were more likely to progress to AD, while those without Aβ pathology did not show the same increased level of hippocampal activation (30), illustrated in Figure 13.4. This link between function imaging and disease led to further pursuit of hippocampal functional connectivity as a potential marker. Allen et al. found that hippocampal functional connectivity was diffusely reduced in subjects with AD, even noting a complete loss of connectivity to the frontal cortices (31). This was expanded upon by Sheline et al. when they showed (Figure 13.5) that the connectivity of a set of regions known as the default mode network (DMN) was reduced even in early (preclinical) AD (32). Although recent work has challenged the ability of DMN connectivity to differentiate those with AD or MCI from healthy controls across multiple sites, suggesting that medial temporal lobe connectivity may be a better marker (33), it is clear that fcMRI possesses the ability to advance our understanding of the disease process, and may assist in differentiating dementia diagnoses (6).
Figure 13.4 The hippocampal baseline activity, as determined by FMRI, was increased in those with Aβ, despite the reduced hippocampal volume (HV). Note that cognitive testing results were similar in the two groups.
Figure 13.5 Areas of altered functional connectivity between AD and PiB negative cognitively normal individuals when using a precuneus (DMN) seed region. Note that a similar pattern was found in PiB positive individuals without cognitive dysfunction when compared to PiB negative individuals. VC = visual cortex. L Hip = left hippocampus. L Parahip = left hippocampus. AC = anterior cingulate. GR = gyrus rectus.
Imaging Neuroinflammation
In addition to the elevated Aβ and hyperphosphorylated tau levels, increases in inflammatory cytokines (IL-1) and activated microglia suggest that neuroinflammation plays a role in AD pathology (34,35). Likewise, neuroinflammation has been implicated in PND (12). Thus, the growing abilities to image neuroinflammation is receiving much attention, with PET imaging via radiolabeled targets of TSPO taking a leading position.
A large, recent study using a fluoride-based TSPO tracer found that microglial activation is present in the prodromal stage for AD, with image intensity correlating with mini-mental status exam scores and gray matter volume (19), hypothesizing that inflammation may play a protective role in the early stages of clinical progression. Although these findings in Figure 13.6 need to be replicated, this study utilized two important advances in TSPO imaging (36) – factoring in whether or not subjects had low, medium, or high binding affinity for the PET marker, and using standard uptake value normalization (as opposed to a two-compartmental method that requires arterial blood sampling). The surprising finding that microglial activation may actually be protective in the early stages of AD would not have come to light without the continual advancements in neuroimaging, and provides solid evidence that neuroimaging can lead to new insights into PND as well.
Figure 13.6 TSPO imaging using standard uptake values (SUVr) correlated with (A) MMSE; (B) gray matter volume in patients with Alzheimer’s disease; and (C) gray matter volume in patients with prodromal Alzheimer’s disease. Note that these correlations were positive after adjusting for age and TSPO genotype.
As another example of successful neuroinflammation imaging use, consider Parkinson’s disease. In Figure 13.7, levels of TSPO tracer are elevated in the pons, basal ganglia, frontal cortices, and temporal cortices in patients with Parkinson’s disease. While this does suggest widespread microglia activation, longitudinal examination suggests that these levels remain the same over a 2 year period (37), meaning that microglial activation may occur early in the disease. Furthermore, the use of an experimental inhibitor of myeloperoxidase (a reactive oxygen generating enzyme expressed by microglia and thought to be involved in the neurodegeneration seen in Parkinson’s disease) was shown to decrease the total distribution volume in a separate TSPO marker (38). Thus, the use of neuroinflammation markers may offer a unique method for monitoring the efficacy of disease treatment (39). For PND, similar design comparing different anesthetic techniques for their effect on markers of neuroinflammation could help in the design of anesthetic plans that protect those at risk.
Figure 13.7 TSPO imaging is also able to differentiate between healthy individuals and patients with Parkinson’s disease. Note the elevated binding in patients in the frontal cortex, basal ganglia, pons, and temporal cortex.
To date, there has been limited, but exciting, neuroimaging studies of anesthesia/surgery-induced neuroinflammation as it relates to PND. Zhang et al. have reported about their experience using a TSPO marker ([18F]-FEPPA) in nonhuman primates (40). After exposing four postnatal day 5 or 6 rhesus monkeys to 70% nitrous oxide and 1% isoflurane for 8 hours, they acquired PET images with [18F]-FEPPA at 1 day, 1 week, 3 week, and 6 month time points. The same was done in four control monkeys without the 8 hour exposure. Increased tracer uptake, presumably reflecting neuronal damage and gliosis, was found in the frontal and temporal cortices at the 1 week time point. The 3 week scans showed that the exposed monkeys’ uptake values tended to be higher, but not statistically significant. Values were equal at 6 months, suggesting that acute neuroinflammation or injury occurs after inhalational anesthetic administration, and that advanced imaging techniques would be able to monitor this process longitudinally.
Interestingly, human TSPO imaging suggests a strong downregulation in brain immune function after surgery, with decreased binding of the TSPO marker [11C]-PBR28. Forsberg et al. found that tracer uptake was decreased 3–4 days postsurgery after prostatectomy and returned to baseline level upon 3 month follow-up scanning, in association with a decrease in the immunoreactivity of peripheral blood cells to lipopolysaccharide stimulation (41). The response of the immune system to surgery is complicated, with transient initial pro-inflammatory environments changed to anti-inflammatory ones with varying time courses in preclinical work. Clearly there is still much to learn about the relationship between inflammation, surgery/anesthesia, and cognitive function.
Neuroimaging in Perioperative Neurocognitive Disorder
Neurologic changes have been recognized to occur after cardiac surgery for quite some time. MRI was used as early as 1991 to assess for “high intensity spots” in the brain before and after surgery (42) in an attempt to understand the source of these changes, although early studies were limited by the MRI technology of the time. As neuroimaging’s abilities have grown, so has the information that has been gained by its application to PND. Below we will summarize the findings as they relate to PND for anatomic and functional imaging methodologies.
White Matter Hyperdensity Imaging after Cardiac Surgery
Initial efforts focused on imaging the effects of ischemia, as this could be caused by the common occurrences of hypoperfusion or embolization from aorta cannulation during cardiac surgery. The most common scanner approaches used for ischemia include diffusion-weighted imaging (DWI), fluid attenuated inversion recovery sequencing (FLAIR), and sequences that exploit differences in the T2 relaxation time of brain tissue (see Figure 13.8 for examples). DWI has the advantage of only showing lesions that are approximately 2 hours to 2 weeks old, so preoperative imaging is not necessary to determine if lesions are new, whereas this is required in FLAIR and other T2-weighted sequences. In support of ischemia, new cerebral lesions often termed white matter hyperdensities (WMH) have been shown to appear in an average of 29% of patients after cardiac surgery (43). Furthermore, in testing of 86 patients after cardiac artery bypass grafting (CABG) surgery, Gerriets et al. found that 15% had new asymptomatic lesions on DWI-MRI and that the occurrence of these correlated with early decreases in letter interference and attention (44).
Figure 13.8 Examples of imaging techniques used to identify areas of ischemia. (A) Normal FLAIR image. (B) FLAIR showing white matter hyperdensities. (C) Normal DWI. (D) DWI image showing acute infarct in the right thalamus.
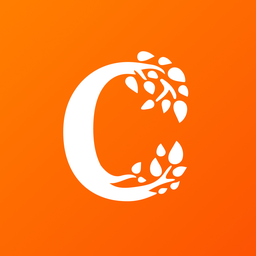
Full access? Get Clinical Tree
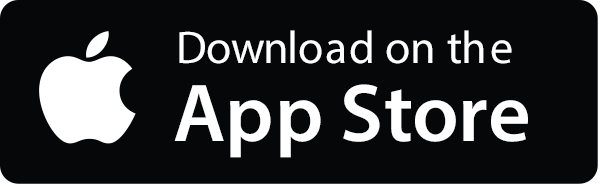
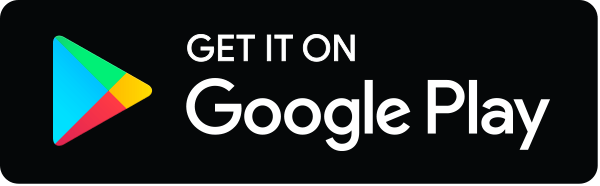
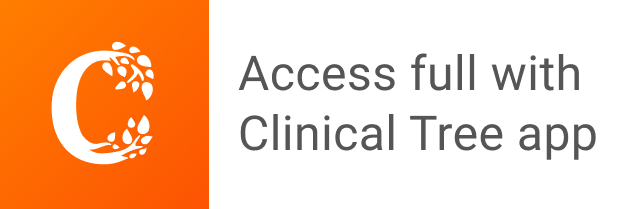