Abstract
Human physiology is well adapted to sea-level atmospheric pressure. Decreased atmospheric pressures at altitude have profound effects on human physiology and anesthetic delivery. Seemingly arcane, the need for high-altitude anesthesia and critical care in disaster response is unusually common. The seismology and meteorology of high-altitude mountainous environments leads to frequent natural disasters. Approximately 140 000 000 people worldwide live persistently at altitudes above 2500 meters (8000 feet). Additionally, millions of people transiently visit high altitudes for vacation hiking, skiing, sport climbing, or other recreational pleasures. These endemic and visiting populations are subject to the risks of natural disasters, in addition to their usual needs for surgical care at elevation. Anesthesiology providers are also asked to support military operations, aviation, mountain-climbing expeditions, and disaster-response humanitarian missions at extreme altitudes. These latter operations may be conducted in minimally developed areas with limited resources and involve high-risk behaviors, trauma, minimal time for acclimatization, and hostile environmental exposure.
Human physiology is well adapted to sea-level atmospheric pressure. Decreased atmospheric pressures at altitude have profound effects on human physiology and anesthetic delivery. Seemingly arcane, the need for high-altitude anesthesia and critical care in disaster response is unusually common. The seismology and meteorology of high-altitude mountainous environments leads to frequent natural disasters. Approximately 140 000 000 people worldwide live persistently at altitudes above 2500 meters (8000 feet). Additionally, millions of people transiently visit high altitudes for vacation hiking, skiing, sport climbing, or other recreational pleasures. These endemic and visiting populations are subject to the risks of natural disasters, in addition to their usual needs for surgical care at elevation. Anesthesiology providers are also asked to support military operations, aviation, mountain-climbing expeditions, and disaster-response humanitarian missions at extreme altitudes. These latter operations may be conducted in minimally developed areas with limited resources and involve high-risk behaviors, trauma, minimal time for acclimatization, and hostile environmental exposure. Expedition climbers and other adventurers have required surgical care at altitude in the past – not without anesthetic adventure and adverse outcome.1 Safe and effective anesthesia care at high altitude requires an understanding of the normal and pathological effects of altitude and an appreciation for the anesthetic challenges in this environment.
Basic Science
Atmospheric pressure has a non-linear decrease as altitude increases. Half of the atmosphere is below 18 000 ft and the remaining half extends up to >100 000 ft. Increased altitudes are often categorized to denote their increasing effect of physiology:
“High altitude” begins at 1500 m (~5000 ft) above sea level.
“Very high altitude” begins at 3500 m (~11 500 ft)
“Extreme altitude” begins at 5500 m (~18 000 ft)
Anesthetics delivered between 5000 and 11 500 ft require only modest changes from standard sea-level practices. Anesthetic care above 11 500 ft becomes increasingly problematic. The distinction and risks of “extreme altitude” are self-explanatory.
Fractional atmospheric oxygen concentration remains approximately 21% at all altitudes. As atmospheric pressure decreases with elevation, the absolute partial pressure of oxygen in air declines according to Dalton’s Law – “The sum of all the partial equals the total pressure.” (Pbar × 0.21) calculates the atmospheric oxygen pressure at any altitude. The alveolar gas equation defines a partial pressure for alveolar oxygen based on barometric pressure. A simplified form of the equation is:

where PAlveolar is the partial pressure of oxygen in the alveolus (mmHg) – “PAO2,” FiO2 is the fractional concentration of oxygen in inhaled gas (21% unless enhanced), PBar is the barometric (atmospheric) pressure (mmHg), 47 mmHg is the vapor pressure of water at body temperature (altitude does not change), PaCO2 is the partial pressure of CO2 in the alveolus (mmHg), and RQ is the respiratory quotient (moles CO2 produced per moles O2 consumed – typically about 0.82 for normal diet).
Using the alveolar gas equation, the effects of altitude and carbon dioxide on alveolar and arterial oxygen become very evident (calculations assume PaCO2 = 40 – no compensation with hyperventilation):2
At sea level – PBar is 760 mmHg, PAO2 is 101 mmHg, and hemoglobin saturation (SaO2) is 98%.
At 5000 ft – PBar is 632 mmHg, PAO2 is 73 mmHg, and SaO2 is 95%
At 10 000 ft – PBar is 522 mmHg, PAO2 is 51 mmHg, and SaO2 is 86%.
At 15 000 ft – PBar is 438 mmHg, PAO2 is 32 mmHg, and SaO2 is 62%.
At 20 000 ft – PBar is 364 mmHg, PAO2 is 17 mmHg, and SaO2 is 24%.
(This last altitude is only sustainable with hyperventilation/hypocarbia or supplemental oxygen.)
With PaCO2 = 20 mmHg – note the effect of hyperventilation on saturation
At 20 000 ft – PBar is 364 mmHg, PAO2 is 42 mmHg, and SaO2 is 78%.
Demographics
All people are subject to the normal physiological effects of altitude. Rapid ascent to high altitude without adequate acclimatization additionally risks altitude-related illnesses. Physiologic adjustment to altitude requires both time and patience.
Adaptation
Acute adaptation to high altitude involves hyperventilation and increased cardiac output. Lower PBar leads to lower PAO2, decreased SaO2, and PaO2. Increased hypoxic ventilatory drive from low PaO2 leads to hyperventilation. Hyperventilation leads to decreased PaCO2 and PACO2, enhancing alveolar oxygen tension (per above illustration). As hyperventilation is the primary means of acute adaptation to ascent, the ability to rapidly tolerate hypobaric environments depends largely on sufficient pulmonary reserve. The degree of hypocapnia can be striking. End-tidal CO2 on Mt Everest has been measured at 7.5 mmHg, clearly low enough to modify cerebral and other organ blood flow. Decreased gas density offsets some of the adverse work of breathing from hyperventilation. Cardiac output is increased by sympathetic signaling due to hypoxemia, particularly through increased heart rate. This acute response may be detrimental to individuals with ischemic or valvular cardiac disease or with pre-existing pulmonary hypertension.
Longer-term adaptation occurs over days to weeks. Mechanisms include:
Increased 2,3-DPG levels due to hypoxic stress, shifting O2–Hgb dissociation curve toward the right and facilitating O2 unloading in tissues
Increased erythropoiesis – polycythemia toward >50% Hct
Increased cardiac output secondary to hypoxemia
Renal compensation for the alkalosis of hypocarbia – largely bicarbonate wasting to improve left-shifted O2–Hgb dissociation curve
Genetic differences modify adaptive responses
The complete mechanism of adaptation is not fully elucidated.
Pathophysiology at Altitude
Both anesthesia providers and their patients arriving acutely at high altitudes are susceptible to hypobaric illnesses. Over-exertion, poor hydration, and young age may contribute to risk. Physical fitness and gender do not seem to affect incidence. Sudden exposure to very high or extreme altitudes (>11 500 ft) can be fatal. Unconsciousness can occur within minutes and death may follow without supplemental oxygen. Although discussion of these illnesses may seem tangential to “high-altitude anesthesia,” these conditions may affect either the provider or the patient. Prolonged emergence, refractory hypoxemia, and other adverse anesthetic patient outcomes may be explained by these mechanisms. Providers impaired by these conditions constitute a substantial hazard to their patients. Cerebral hypoxia impairs judgment – perhaps your judgment!
Acute high-altitude exposure can cause several forms of illness, overlapping in both pathophysiology and clinical presentation:
acute mountain sickness (AMS)
high-altitude pulmonary edema (HAPE)
high-altitude cerebral edema (HACE).
Acute Mountain Sickness
AMS is the mildest mild form of hypobaric illness.
Symptoms
Early symptoms (12–24 hours): headache refractory to standard analgesics, nausea, anorexia, lassitude, sleep disturbances, peripheral edema. Judgment may be impaired.
Late symptoms: can progress to shortness of breath, intense snoring, vomiting, hallucinations, and severely impaired cognitive function.
Advanced symptoms: severe dyspnea, cyanosis, decreased SaO2, ataxia.
Treatment
If severe, definitive treatment is descent! Often descent of only 500–1000 m leads to complete resolution of symptoms. Most symptoms resolve in several days, even at altitude.
Rest, hydration, analgesics, oxygen all can help.
Acetazolamide 250 mg q 8–12 hours improves symptoms and SaO2 (especially during sleep).
Dexamethasone 4 mg q 6 hours.
Prevention
Ascend slowly (not always possible in military or disaster operations)
Oxygen, particularly at night while sleeping
Daily altitude gain of no more than 300 m above 3000 m
After ascending 1000 m spend two consecutive nights
Rest on arrival at altitude, avoiding over-exertion, adequate hydration
Acetazolamide 250 mg q8 hours beginning at least 24 hours before ascent and continued for two to three days after reaching highest altitude.
Two additional forms of altitude-induced illness are, though less common, more severe and life threatening.
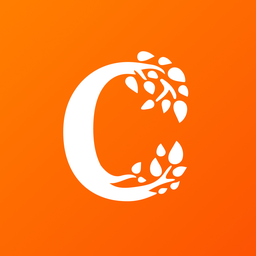
Full access? Get Clinical Tree
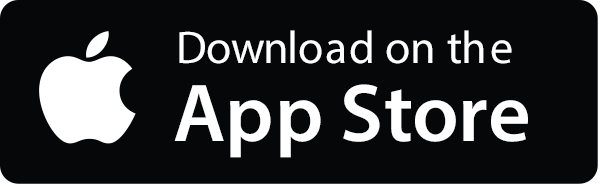
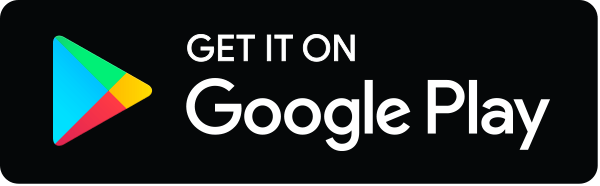
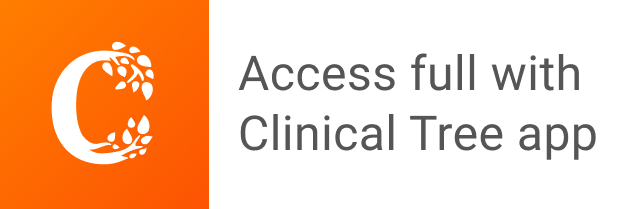