Abstract
Novel tools in neuroscience (genetic, optical, pharmacological) are being used to advance our understanding of the complexity and functioning of the brain, the primary target of general anesthesia.
Anesthesiologists administer drugs to facilitate procedures that dramatically alter brain function on a daily basis to create loss of consciousness, amnesia, analgesia and immobility. Novel neuroscientific approaches are targeted towards decoding consciousness and memory, and are thereby contributing to our understanding of the mechanisms of anesthetic actions. This chapter reviews the neuroscientific understanding of the brain and the technical approaches leading to these discoveries. After a brief review of the major milestones in brain research, we describe the cellular mechanisms that govern neuronal activity as well as the neurophysiological basis of global network activity. The major structures of the brain and their neurophysiological roles as they pertain to anesthesia are then described.
Keywords
Neuron, Synapse, Action potential, Neural network, Brain structure
Chapter Outline
The Brain: Structure Determines Function
The neuron is the fundamental unit of information processing and transfer in the central nervous system (CNS). It is, however, the collective activity of hierarchically organized neuronal circuits and networks that underlies its behavioral and homeostatic functions. These functions are emergent properties of a complex system. Therefore the CNS has to be examined on different organizational levels: properties characteristic of a functional unit on one level of organization are absent from lower-level elements and cannot predict properties of a higher-level unit. How exactly behavior emerges from the activity of individual neurons, circuits, and networks is a question at the cutting edge of research.
Individual neurons and/or their organelles carry the primary molecular targets of anesthetic drugs. Current thinking attributes most of the desirable effects of general anesthetics to the modulation of membrane proteins. Drug-induced alterations in the function of many voltage-, transmitter-, and otherwise gated channels are relatively well characterized. By contrast, how these alterations propagate through increasing levels of complexity to finally result in sedation, amnesia, hypnosis, and immobility is less well understood. General anesthetics also affect second-messenger systems either directly or via effects on G-protein–coupled receptors and mitochondria. The significance of the latter effects for anesthesia is unclear.
Historical Perspective
The brain: ancient Egyptians tossed it when preparing the body for the journey into the afterworld. Aristotle thought it a radiator to cool the heart. But more than 2000 years and dozens of Nobel Prizes later, the American Society for Neuroscience alone counts more than 35,000 members, many of whom devote their lives to its study.
Except for the last decade of the 19th century, brain science in the 1800s was dominated by physicians such as Broca, Wernicke, Fritsch, and Brodmann. They and others started the process of a functional localization of the brain. Pavlov linked psyche to behavior and learning with the development of the concept of the conditioned reflex, which is still widely used in neuroscience today. His achievements were honored with the Nobel Prize in 1904. In 1906, Golgi shared the 1906 Nobel Prize with Ramón y Cajal, two central protagonists in the grand debate on the cellular versus reticular organization of the brain. The debate was settled in favor of Cajal’s view that discrete cells as opposed to a reticulum formed the structure of the brain. However, as nature is not doctrinaire, some neurons (and parts of the glia) do act syncytially by maintaining specialized intercellular channels, common during embryogenesis, into adulthood. In 1897, Sherrington postulated communication via the synapse on theoretical grounds. This turned out to be a fundamental characteristic of the nervous system, which however, was incontrovertibly proven only decades later with the aid of electron microscopy.
The 20th century witnessed an exponential growth of science as a collaborative endeavor. Therefore individual names, no matter how outstanding, give progressively less and less justice to all those who contribute to important advances in science. Nevertheless, we use the names of Nobel Prize laureates to anchor a flash review of concepts particularly relevant to this chapter. In 1915, while held in a Russian prisoner of war camp, Bárány received the Nobel Prize for his studies of the physiology and pathology of the vestibular system. In 1932, Adrian and Sherrington shared the Nobel Prize for their contributions to neuronal signal propagation. Dale and Loewi shared the Prize in 1936 for work on transmitter release, but Dale’s principle (which states that each neuron releases only one type of neurotransmitter) was proven false by the end of the 20th century. Nevertheless, it underlies the familiar classification in widespread use today (e.g., adrenergic, gamma-aminobutyric acid [GABA]ergic). Hodgkin and Huxley shared the Nobel Prize with Eccles in 1963 for discovering the ionic mechanisms involved in excitation and inhibition of the nerve cell membrane. Their biologic model—the giant axon of the Atlantic squid—was so central to this quantum leap in understanding that a contemporary remarked that Loligo pealei should have been awarded the prize. Axelrod, von Euler, and Katz shared the prize in 1970 for work on neurotransmitter storage, release, and inactivation in the axon terminal. The Nobel Prize to Hubel, Wiesel, and Sperry in 1981 marked a shift in neuroscience toward a comprehensive, system-level understanding of the brain that remains the frontier of neuroscience today. Hubel and Wiesel suggested a hierarchical model for the identification of complex visual themes. Sperry was awarded the prize for his work on lateralized specialization of brain function. Advances in our understanding of the mammalian brain’s navigation system were recognized by the 2014 Nobel Prize to O’Keefe and the Mosers underlining the increased attention of the neuroscience community to system- and network-level approaches.
Glia
Glial cells (or neuroglia: “nerve glue”) are nonneuronal cells that outnumber neurons and are ubiquitous in the CNS. Glial cells take up, metabolize, respond to, and release neurotransmitters and modulators; control the microenvironment that surrounds neurons; and support communication. Glial cells also play a number of other roles in CNS homeostasis. However, glial cells are not known to actively transfer and process discrete information.
Two basic types of neuroglial cells can be distinguished: macroglia (oligodendrocytes, astrocytes) and microglia . Oligodendrocytes fulfill in the CNS the role that Schwann cells play in the peripheral nervous system with some adaptations. To conserve space, one oligodendrocyte cell body myelinates multiple axons and the myelin itself consists of a single sheet of oligodendrocyte plasma membrane without cytoplasm wrapped tightly around an axonal segment. Each myelinated segment is termed an internode because of the bare segments of axon present at each end of it—the node of Ranvier. The action potential can leap from node to node—a process known as saltatory conduction, allowing rapid conduction even along thin axons.
Astrocytes constitute 20% to 50% of the volume in most brain regions. Two main forms predominate: protoplasmic in the gray and fibrous in the white matter. Astrocytes isolate the brain from the rest of the body by covering the surface of capillaries and ensheathing the nodes of Ranvier, synapses, and dendrites with extensions of their bodies, creating the blood-brain barrier. Astrocytes are connected to each other by gap junctions forming a syncytium that facilitates the diffusion of small molecules across the CNS. They also communicate with neurons via calcium ion (Ca 2+ )-induced transmitter release. Astrocyte precursors (radial glia) guide neuronal migration in early development. Throughout life, astrocytes synthesize a plethora of growth factors and are the major source of extracellular matrix proteins and adhesion molecules.
Microglial cells are immune-competent cells, termed the macrophages of the CNS . They are the least understood cell type of the CNS even though they account for a substantial fraction of all cells (5%-20% in the mouse brain; human white matter has more microglia than its rodent counterpart).
Glial cells are not known to be materially affected by anesthetics in a way that can be linked to acute behavioral changes.
CSF, Interstitial Fluid, and the Glymphatic System
Over the past decades renewed interest in certain peculiarities of brain physiology, especially its immune-privileged status and the puzzling lack of a lymphatic system, has led to a series of elegant experiments that have added cutting-edge experimental techniques to older observations and are redefining important aspects of brain physiology with important implications for pathophysiology.
The brain’s high metabolic rate (the average neuron is estimated to consume 4.7 billion adenosine triphosphate [ATP] molecules per second) results in proportional waste accumulation in the interstitial fluid. While the density of lymph vessels outside the brain correlates with the rate of tissue metabolism (lymph flow is essential for clearing waste from metabolically active tissues), the complete lack of conventional lymphatic vessels has been a conundrum of CNS physiology. Related to the problem of waste disposal is the question of how the CNS fulfills the immunologic functions, which in the periphery, are carried by the flow of interstitial fluid and immune cells through the lymphatic vessels and lymph nodes.
The standard model postulates that small molecules and hydrophobic compounds move across the blood-brain barrier relatively unrestrictedly and specific blood-brain barrier transporters readily clear other molecules from the brain. For compounds not falling into these two categories, diffusion by way of the interstitial fluid to CSF-containing spaces (ventricles, subarachnoid space) was assumed to suffice. Clearance from the cranium was accomplished by CSF reabsorption into the dural sinuses via the arachnoid granulations and into peripheral lymphatic vessels of the nasal mucosa and neck along perineural spaces surrounding the cranial nerves. An important weakness of the model, however, is that the distances between much of the brain tissue and the CSF compartments are far too great for efficient clearance by simple diffusion, particularly for large molecules with low diffusion coefficients (such as peptides and proteins).
Recent research is providing a coherent, albeit not yet exhaustive, solution to these issues. Contrary to previously held views, the new model postulates that CSF is produced not only in the choroid plexus but also by filtration of water through capillary walls governed by hydrostatic and osmotic forces. CSF flows from the subarachnoid space along a system of perivascular tunnels known as the Virchow-Robin spaces ( Fig. 8.1 ). These perivascular spaces act as low-resistance paths for CSF influx. Endfeet of astrocytes ensheathe the brain vasculature forming tunnels around the vessels. Fluid from these tunnels is taken up by aquaporin 4 water channels, which are expressed in a highly polarized manner in astrocytic endfeet and transferred into the interstitial fluid of the dense brain parenchyma. Influx of CSF on the arterial/capillary side of the circulation drives the flux of interstitial fluid toward the perivenous spaces surrounding the large veins. The interstitial fluid then drains out of the brain into peripheral lymphatic vessels into the deep cervical lymph nodes as described in the standard model. Acknowledging the central role of glia, the term “ glymphatic” has been coined to describe this system. Moreover, while it remains accepted that no lymphatic vessels in the classic sense exist within the brain and spinal cord parenchyma, a network of lymphatic-like vessels has been identified in the dura mater of the mouse brain. Endothelial cells in these vessels share similarities with conventional lymphatics (e.g., expression of traditional markers of tissue lymphatic endothelial cells) but with distinct adaptations to their function in the CNS. Meningeal lymphatics enable the drainage of the macromolecules and immune cells originating from the CNS to the deep cervical lymph nodes (see Fig. 8.1 ). Remarkably, genetic ablation of this system did not lead to increased intracranial pressure, indicating the existence of separate specialized systems for CSF volume regulation (arachnoid villi, cribriform plate, perineural spaces) and immune surveillance (meningeal lymphatics).

Intriguingly, the glymphatic system functions mainly during sleep and appears to be largely quiescent during wakefulness. The biologic need for sleep across all species may therefore reflect the necessity to enter a state of activity that enables elimination of potentially neurotoxic waste products (including β-amyloid, a mediator of Alzheimer disease). Flux through the glymphatic system is energy-consuming and assisted by arterial pulsatility. It diminishes with age and undergoes changes associated with a number of conditions affecting the CNS (e.g., traumatic brain injury, neurodegenerative diseases, and diabetes mellitus). The effect of anesthetics on flux through this system is unknown with the exception of ketamine-xylazine anesthesia, which increases flux similarly to sleep.
In addition to waste elimination, the glymphatic system also facilitates the brainwide distribution of glucose, lipids (including cholesterol and its degradation products), amino acids, growth factors, neuromodulators, as well as β-amyloid.
The Neuron
The neuron is the fundamental building block of the nervous system. Neurons are diverse in nature, interconnected, adaptive, and therefore, as ensembles, give rise to the stunning complexity of the brain. A complex system is endowed with emergent properties —that is, properties that appear only in ensembles as opposed to being present fully in the individual element. The human brain contains more than 10 11 neurons. Their cell bodies are located in gray matter areas of the CNS, whereas the white matter consists of fibers connecting neurons from different regions.
Neurons are excitable cells. This property is harnessed to receive, process, integrate, and transmit signals. Their structure is optimized to integrate multiple inputs and deliver what formerly was considered an all-or-none (binary), digital signal in the form of an action potential to other, potentially distant, elements. Action potentials, however, should not be interpreted as isolated events where information transfer is concerned. Both their rate modulation and their phase relationship with respect to ongoing oscillations encode information. Neurons in the CNS are connected by approximately 10 14 synapses, which are specialized structures allowing information transfer and hence networking.
Basic Structure
The generic neuron contains three basic elements: dendrites (signal reception and processing), soma or cell bodies (signal integration and cellular housekeeping apparatus), and axons (information output). These three elements vary in complexity, shape, and length, giving rise to a dazzling variety of neuronal morphology ( Fig. 8.2 ).


The soma is the part of a neuron that is most reminiscent of a conventional cell. It contains all the standard cellular components found in other cells, such as nucleus, protein production (ribosomes), and modification machineries and energy generators (mitochondria). The soma also undergoes neuron-specific long-term changes during development, plasticity, degeneration, and aging. In addition, the soma is the hub linking signal input to information output.
The dendrites are elongated cytoplasmic processes. Usually a neuron has more than one dendrite. Each dendrite bifurcates multiple times, creating a dendritic arbor in the vicinity of the soma; it can stretch to other cortical layers but does not spread to distant regions of the brain. The dendritic arbor creates a large, intricately organized membrane surface area to receive input from other neurons. The arbor hosts vast numbers of the postsynaptic component of chemical synapses—transmitter receptors—targeted by incoming axon terminals that form the presynaptic side. A typical pyramidal cell (e.g., in the hippocampus or in cortical layer V) receives many thousand synapses: excitatory inputs on dendrites, inhibitory on soma and dendrites. The structure of the dendritic tree and the location of each synapse on it determine the nature of input summation and local integration and hence their relative strength. Spines are small processes of varying shapes found on many dendrites. These processes are specialized sites of synaptic connections, increasing the dendritic membrane and subdividing the subsynaptic structures ( Figs. 8.3 and 8.4 ). Spines are highly dynamic and can change their size and composition on a time scale of minutes in response to neuronal activity, thereby creating, changing, or eliminating synaptic points of contact.


The axon is a very thin cytoplasmic process that carries the output signal of the neuron (the action potential) to its targets. Typically, a single axon originates from and terminates in specialized structures termed axon hillocks at the soma and presynaptic terminals at its destination. Normally, it bifurcates multiple times on the way and creates an axonal arbor connecting with many other neurons. Axonal lengths range from less than a millimeter (local interneurons) to more than 1 m (spinal α-motoneurons).
Axons are usually much longer and thinner than dendrites; hence they require mechanisms that enable them to reliably and efficiently transfer information over long distances, supporting the presynaptic machinery in the terminal at the target while maintaining their own structural integrity. The myelin envelope and the axonal transport system are two mechanisms to this end.
Myelin is a composite of multiple layers of lipid membranes formed by the oligodendroglia in the CNS (Schwann cells in the peripheral nervous system). Myelin electrically insulates the axon, enabling high-speed propagation of action potentials with minimal energy expenditure (varying with the degree of insulation).
Axonal transport is a “housekeeping” mechanism that moves vesicles and proteins from their site of synthesis in the soma along the whole length of the axon, and reverse transport relays signals back to the soma. It is an active, energy-consuming process using the cytoskeleton (e.g., microtubules) to meet the metabolic needs of the axon, its collaterals, and the presynaptic terminals because diffusion alone would not suffice.
Excitability
Excitable cells are characterized by the ability to dynamically change the electrical potential across their cell membrane. Typical examples are muscle cells and neurons. Neurons use this property to integrate and disseminate information with precise and independent control of excitability in different functional compartments. Alterations in excitability are an important aspect of anesthetic drug action.
A living cell is defined by its ability to remain segregated from its environment. This fundamental property is made possible by the selective permeability of the cell membrane (the lipid bilayer and the proteins embedded into it). The intracellular ions are dominated by potassium ions (K + ), while extracellularly high sodium ions (Na + ) and chloride ions (Cl − ) hark back to the ionic composition of sea water ( Table 8.1 ). Because of its hydrophobic interior, the lipid bilayer prevents charged molecules and most ions from crossing the membrane. By contrast, water and other small uncharged molecules (e.g., urea) diffuse freely. Membrane-spanning proteins selectively control the movement of ions and other small molecules across the membrane, rendering this lipid envelope semipermeable. Membrane transport systems can be divided into two principal categories: catalytic and stoichiometric. The proteins responsible for this property are traditionally grouped into pores and channels (catalytic) on one hand and pumps and transporters (stoichiometric) on the other. Catalytic systems carry out movement only “downhill”—that is, toward the electrochemical equilibrium—while stoichiometric systems can carry out transport “uphill” —away from the equilibrium with energy expenditure. Cells also have special mechanisms to transport large molecules across the cytoplasmic membrane. Excitable cells in particular are endowed with a rich assortment of ion channels.
Ion | Intracellular Concentration (mm) | Extracellular Concentration (mm) | Steady-State Potential at 37°C (mV) |
---|---|---|---|
Na + | 10 | 145 | +70 |
K + | 140 | 5 | −87 |
Ca 2+ | 10 −4 | 1.5 | +125 |
Mg 2+ | 6.5 | 2 | −15 |
Cl − | 4 | 110 | −86 |
HCO 3 − | 12 | 25 | −19 |
The differential distribution of ions inside and outside the cell creates chemical concentration gradients. For example, the concentration of K + is high inside and low outside the neuron. This creates a tendency for K + ions to diffuse out of the cell through K + channels that are typically open at the resting membrane potential (RMP) . Because K + is positively charged, this creates a redistribution of electrical charge, with the inside of the cell becoming more negative because the membrane is impermeable to large molecules like negatively charged proteins. The excess negative charge inside the cell attracts K + ions and, at some point, the tendency to diffuse out of the cell along the chemical concentration gradient will be offset by the electrical attraction from inside the cell, reaching electrochemical equilibrium. This equilibrium is dynamic as ions continuously cross the membrane while the net flow of charge is zero. The relation between an ion’s concentration gradient and the electrical gradient it creates was described in the 19th century by Nernst in his classic equation:
E = R T z F ln ( [ I o n ] o u t [ I o n ] i n ) ,
Because most of the values in the Nernst equation are constant under biologic conditions, the equation can be simplified assuming intracellular and extracellular K + concentrations of 140 and 5 mmol/L at 37°C as:
E = 60 log ( [ K o u t + ] [ K i n + ] ) = 60 log ( 5 140 ) = − 86.8 m V
This means that, at −86.8 mV and the K + concentrations given above, no net flow of K + occurs even if the membrane is freely permeable to K + . Similarly, for each ion there is a membrane potential at which its concentration gradient will be balanced by its electrical gradient. These equilibrium potentials become significant determinants of the membrane potential when opening of appropriate channels renders the membrane permeable to a specific ion (e.g., Na + ). Table 8.1 lists the Nernst potential of some common ions that influence the neuronal membrane potential.
The membrane potential of a cell is determined by the gradients of all the intracellular and extracellular ions, scaled in proportion to their permeability through the membrane: the greater the permeability of the cell membrane to a specific ion, the stronger its effect on the membrane voltage. This is described by the Goldman-Hodgkin-Katz equation :
E = R T F ln ( ∑ i N P C i [ C i ] o u t + ∑ j M P A j [ A j ] i n ∑ i N P C i [ C i ] i n + ∑ j M P A j [ A j ] o u t ) ,
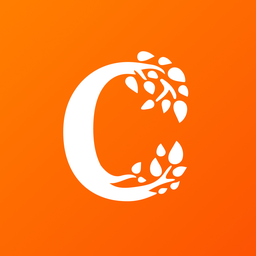
Full access? Get Clinical Tree
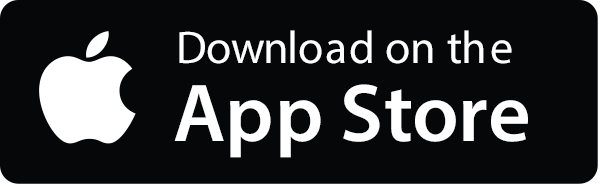
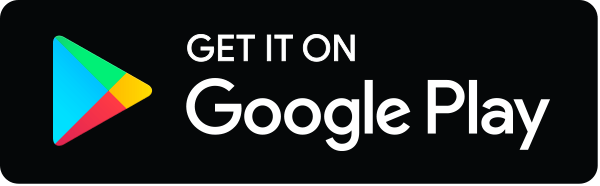