Most neurologic emergencies that require the intervention of an anesthesiologist are caused by head and spine trauma, leading to traumatic brain injury (TBI) and traumatic spinal cord injury (SCI). NonTBIs which can cause acute patient instability include nontraumatic intracranial hemorrhage, ruptured cerebral aneurysms or arteriovenous malformations, acute arterial hypertension, or chronic anticoagulation therapy; acute hydrocephalus; intracranial tumors with impending brain herniation; and ischemic stroke. Likewise, tumors or hematomas compressing the spinal cord may cause acute spinal cord injury.
The aims of the acute care of patients with either brain injury or SCI are stabilization of the patient and prevention of secondary neurologic injury. Secondary insults to other organs and systems as a result of the primary neurologic injury and coexisting injuries in patients with trauma contribute to development of the secondary injury of the neurologic system, adversely affecting outcome.
This chapter focuses on the acute care of the neurologically unstable patient with brain and spinal cord injuries, regardless of the cause. We discuss the initial neurologic evaluation, the evaluation of other organ systems, and the goals of the acute care of the unstable patient.
Brain injury
Initial Evaluation
Every patient with trauma should be approached in accordance with the basic Advanced Trauma Life Support (ATLS) principles that prioritize systems assessment in an order of ABCD: A—airway, B—breathing, C—circulation, D—disability/ neurologic assessment, E—exposure.
A basic neurological assessment, known by the mnemonic AVPU (Alert, Verbal stimuli response, Painful stimuli response, or Unresponsive), should be performed with the primary survey of patients’ assessment.
A more detailed neurological evaluation is performed at the end of the primary survey and involves assessment of level of consciousness, pupil size and reaction, lateralizing signs, and spinal cord injury level.
The Glasgow Coma Scale (GCS) is a quick method to determine the level of consciousness. An altered level of consciousness requires an immediate re-evaluation of the patient’s oxygenation, ventilation, and perfusion. If factors such as drugs, including alcohol and hypoglycemia, are excluded, a decrease in the level of consciousness should be considered to be due to TBI until proven otherwise.
Every patient with an acute onset of focal neurological deficit, on the other hand, should be considered to have a cerebral vascular accident, until proven otherwise.
Assessment of breathing and circulation with basic vital signs measures should be done concomitantly with the initial assessment of consciousness.
Detailed Neurologic Evaluation
The Glasgow Coma Scale (GCS) score ( Box 10.1 ) comprises the best verbal response, the best motor response, and the presence of eye opening, using a scale from 3 to 15. The scaling system is effective because it is easy to use, has good interobserver reliability, helps guide diagnosis and therapy, and has prognostic significance. Morbidity and mortality are closely related to the initial GCS score, with lower scores predicting a worse outcome, irrespective of the cause of the brain injury. Another factor that predicts the prognosis of the brain injury is age, with a better prognosis noted among pediatric patients, and worse prognosis in adults older than 40 years. Pupillary responses and gag reflexes should be evaluated in the initial neurologic examination. In the acute setting, examination of the size and reactivity of the pupils is particularly important ( Box 10.2 ). A dilated, unresponsive (“blown”) pupil may be a sign of ipsilateral uncal herniation, in which the medial aspect of the temporal lobe (uncus) herniates through the tentorium, thereby compressing the midbrain and nucleus of the third cranial nerve. Bilateral pupillary dilation may be due to bilateral uncal herniation or injury (eg, ischemic or metabolic) to the midbrain. Local eye trauma or third nerve compression may cause a dilated, nonreactive pupil in the absence of a brain injury. In head-injured patients with systolic blood pressure (SBP) greater than 60 mmHg, clinical signs of tentorial herniation or upper brainstem dysfunction are valid indicators of possible mechanical compression. However, in patients with SBP less than 60 mmHg or with cardiac arrest, pupillary signs are unreliable indicators of mechanical compression.
Glasgow Coma scale score (points):
Eye opening:
Spontaneous (4)
To speech (3)
To pain (2)
None (1)
Best verbal response:
Oriented (5)
Confused (4)
Inappropriate (3)
Incomprehensible (2)
None (1)
Best motor response:
Obeys commands (6)
Localizes pain (5)
Withdraws (4)
Flexion to pain (3)
Extension to pain (2)
None (1)
Pupillary size and reactivity
CT scan:
Mass lesion
Cerebral edema
Midline shift/absent basal cisterns
- 1.
The pupil is considered “dilated” if pupillary diameter is > 4 mm.
- 2.
The pupil is considered “fixed” in the absence of constrictor response to bright light.
- 3.
Bilateral pupillary light reflex should be assessed and used as a prognostic factor.
- 4.
The duration of pupillary dilation and fixation should be documented.
- 5.
Any asymmetry of pupils should be documented.
- 6.
Hypotension and hypoxia should be corrected before pupillary assessment.
- 7.
Orbital trauma should be excluded.
- 8.
Pupils should be reassessed after surgical intervention (eg, evacuation of hematoma).
If the patient is comatose (eg, no eye opening, verbal response, or ability to follow commands), after an initial radiologic evaluation is completed, evaluation of midbrain and brainstem reflexes (eg, pupillary response, corneal reflexes, oculomotor movements, and gag reflex) may aid in localization of the injury.
Neuroimaging
After clinical neurologic evaluation and initial stabilization, radiologic evaluation with computed tomography (CT) is performed to diagnose the underlying disease process. If the patient is hemodynamically unstable with intra-abdominal or intrathoracic bleeding, the head CT scan is delayed until the life-threatening surgical bleeding is controlled. If the physical examination indicates a high likelihood of a brain injury, an intracranial pressure (ICP) monitor may be placed concurrently with the laparotomy or thoracotomy. Intracranial mass lesions that require rapid surgical treatment, such as epidural, subdural, or large intracerebral hemorrhages, are readily identified on CT scan ( Figs. 10.1 and 10.2 ). Nonsurgical lesions, such as cerebral edema and hemorrhagic contusion, are also identified ( Fig. 10.3 ). Diffuse cerebral swelling may develop after head trauma, especially in children. The severity of the brain injury can be correlated with the magnitude of the midline shift (see Fig. 10.2 ) and compression of the basal cisterns (see Fig. 10.3 ). In one study, patients with GCS scores of 6 to 8 in whom initial CT scan showed compression of the basal cisterns had a fourfold higher risk of poor outcome than those with normal cisterns. Because patients may often have delayed neurologic deterioration, a repeat CT scan is indicated after any deterioration in neurologic status. Of patients whose conditions deteriorated after a mild head injury, 80% had a mass lesion that potentially required surgery. In contrast, cerebral swelling is more likely to be the cause of deterioration in patients with severe head injury. Occasionally, manifestation of an intracerebral hematoma after TBI is delayed.



Patients with stroke might require an emergency brain MRI to differentiate brain ischemia from bleeding, and subsequent CT angiography, contrast-enhanced CT or MRI to identify brain lesions.
Evaluation of Other Organ Systems
In addition to the neurologic evaluation, an evaluation of other organ systems should be performed ( Box 10.3 ).
- 1.
Respiratory system:
- a.
Upper airway obstruction, inability to protect airway.
- b.
Increased pulmonary shunting.
- c.
Neurogenic pulmonary edema.
- d.
Associated pulmonary injuries: atelectasis, aspiration, pneumothorax, hemothorax, flail chest, pulmonary contusion.
- a.
- 2.
Cardiovascular system:
- a.
Sympathetic nervous system overactivity.
- b.
Hemorrhagic shock.
- c.
Cushing response (hypertension, bradycardia).
- d.
Hypotension (another cause should be sought).
- a.
- 3.
Musculoskeletal system:
- a.
Cervical spine injury in 10% of cases.
- b.
Long bone or pelvic fractures.
- a.
- 4.
Gastrointestinal system:
- a.
“Full stomach.”
- b.
Blood alcohol levels.
- c.
Possible intra-abdominal injury.
- a.
- 5.
Other systems:
- a.
Disseminated intravascular coagulation.
- b.
Hypokalemia.
- c.
Hyperglycemia.
- d.
Diabetes insipidus.
- e.
Hyponatremia.
- a.
Respiratory System
Following assessment of the pattern of breathing and auscultation of the lungs, chest radiography should be performed soon after the trauma patient arrives in the emergency department and in patients with hypoxemia. Many patients with brain injuries are hypoxemic regardless of the mechanism of injury, and an increased degree of pulmonary shunting is associated with a worsened neurologic outcome. Hypoxemia may be due to airway obstruction, hypoventilation, atelectasis, aspiration, and associated lung injuries in trauma patients such as pneumothorax, or pulmonary contusion. On rare occasions, neurogenic pulmonary edema may occur, often in the more devastating injuries. Neurogenic pulmonary edema has been reported after a variety of central nervous system insults, including subarachnoid hemorrhage (SAH), intracranial hemorrhage (ICH), TBI, spinal cord trauma, acute hydrocephalus, colloid cyst of the third ventricle, seizures, and hypothalamic lesions. An acute rise in ICP often, but not always, accompanies the development of pulmonary edema. Increases in ICP may elicit only the sympathetic activation and cardiopulmonary responses that are essential for the development of edema. The mechanism of neurogenic pulmonary edema is not completely understood. Marked sympathetic activation at the time of the injury may damage the pulmonary capillary endothelium by both hydrostatic and increased permeability mechanisms. Increased pulmonary shunting may also be observed in patients with head trauma in the absence of distinct pulmonary edema or pathologic condition. The increased alveolar-arterial oxygen tension gradient in these patients may be related to airway closure caused by a decreased functional residual capacity in a comatose patient or by neurogenic alterations in ventilation–perfusion matching.
Cardiovascular System
Severe brain injury activates the autonomic nervous system and causes a hyperdynamic cardiovascular response consisting of hypertension, tachycardia, increased cardiac output, and electrocardiogram changes that may mimic myocardial ischemia.
A Cushing response, in which bradycardia accompanies the hypertension, may occur. This response is thought to occur because marked intracranial hypertension causes medullary ischemia as a result of decreased cerebral perfusion pressure (CPP) and brainstem distortion, resulting in activation of medullary sympathetic and vagal centers. Although bradycardia accompanies the hypertension in a classic Cushing response, the presence of a relative tachycardia in a patient with a “blown” pupil may indicate that the patient is hypovolemic. If the patient has outright hypotension, other sources of blood loss (eg, pelvic, thoracic, abdominal) should be sought. An isolated, mild-to-moderate TBI is not generally associated with hypotension because the blood loss from the head wound is usually insufficient to cause hypotension in adults.
Acute brain injury may lead to development of a Takotsubo stress cardiomyopathy (stunned myocardium) characterized by an acute transient ST-elevation left ventricle dysfunction, not related to coronary artery disease, and may be easily confused with an acute myocardial infarction. Transthoracic echocardiography (TTE) should be urgently performed to differentiate from coronary artery disease as it demonstrates classical left ventricular dysfunction with akynesis of apex (ballooning) and hypokynesis of other left ventricle segments. Although the pathophysiology of the phenomenon is not exactly clear, it is believed that dramatic catecholamine release with stress, pain or intracranial bleeding lead to so-called “catecholamine cardiac toxicity,” and, possibly, transient coronary spasm, leading subsequently to global, but transient, heart dysfunction. Most frequently (in about 90% of cases) Takotsubo cardiomyopathy is observed in middle-aged female patients with SAH. On occasion, it can also develop in a variety of CNS injuries such as TBI, ICH, ischemic stroke, and epilepsy. Awake patients may present with classic chest pain and shortness of breath, cardiac enzyme elevation, and ST-segment elevations and negative T waves in anterolateral wall on the EKG. Takotsubo cardiomyopathy can be complicated by profound hypotension, arrhythmias including torsade de pointes, heart failure and ventricular rupture and should be treated symptomatically. Usually, an acute cardiomyopathy resolves within a few weeks, echocardiogram normalizes in 6 weeks and ECG in 10 weeks.
Musculoskeletal System
Lateral cervical spine (C-spine) radiography should be performed immediately because approximately 10% of patients with head injuries have associated cervical spine injuries. The lateral cervical spine film picks up approximately 80% of cervical spine fractures and can display lethal injuries such as atlanto-occipital separation. The remainder of the spine series that is required to “clear the neck” (confirm absence of vertebral injuries) should be performed later, after complete evaluation of the head injury. Many patients with head trauma also have long-bone or pelvic fractures that may cause significant blood loss or fat emboli.
Gastrointestinal System
Every patient with a neurosurgical emergency should be assumed to have a full stomach and to be at risk for aspiration. Patients with acute head trauma may also have intra-abdominal injuries. Delayed gastric emptying may persist for several weeks after severe head injury. Significant blood alcohol levels have been found in more than 50% of head-injured patients.
Coagulation
Patients with head trauma may also have disseminated intravascular coagulation, possibly caused by release of brain thromboplastin into the systemic circulation. Outcome is poorer in patients in whom the condition develops. An increase in fibrin split products may identify patients with head injury who are at high risk for adult respiratory distress syndrome. Coagulation factor levels should be checked in the emergency department, and aggressive replacement of platelets and clotting factors may be required. Use of recombinant factor VII has been advocated in patients with polytrauma that includes TBI , and with spontaneous intracranial hemorrhages. However, data are inconsistent regarding mortality benefit, reduction in transfusion requirements, and associated complications from the use of this agent. Given the serious risk of thromboembolic events, recombinant factor VII cannot be recommended as prophylactic therapy in patients without acute bleeding. Prothrombin complex concentrate (PCC), consisting of factors II, VII, IX, and X, and proteins C and S, is a newer agent that has demonstrated superiority to FFP in the rapid reversal of warfarin-associated coagulopathy. , Its use has been expanded beyond warfarin reversal and has been shown to be effective in treating both acquired and induced coagulopathy in patients with TBI. Additionally, PCC offers significant cost savings over recombinant factor VII , and should be considered as a viable alternative for the treatment of coagulopathy in the setting of TBI.
According to the recently issued by ASA/AHA Guidelines for the Management of Spontaneous Intracerebral Hemorrhage (ICH), patients with severe anticoagulation factor deficiency or thrombocytopenia should receive appropriate replacements of factors or platelets. An effect on outcome of platelet transfusion in patients receiving anti-platelet medications, particularly dual antiplatelet medications combining aspirin with P 2 Y 12 -ADP inhibitors (clopidogrel, prasugrel, ticagrelor), is not clear and considered a Class IIB recommendation. In cases of oral anticoagulant-associated intracranial hemorrhage, normalization of coagulation by reversing anticoagulant should be performed as soon as possible. In patients receiving warfarin, intravenous vitamin K (5–10 mg) should be given along with FFP (20 mL/kg) or PCC (20–40 IU/kg) to normalize prothrombin time. Administration of PCC (either 3 or 4 factors) may be advantageous in patients in whom fluid overload should be avoided (eg, patients with heart failure) as about 1 liter of FFP is required to normalize coagulation (10–15 mL/kg of FFP raises level of factors by 10–20%). Patients receiving direct thrombin inhibitor dabigatran or factor Xa inhibitors (rivaroxaban and apixaban) may be treated similarly to patients on warfarin with FFP or PCC as there are no reversal agents available. An antibody fragment idarucizumab has been recently advocated for the effective reversal of dabigatran; its safety in neurosurgical patients, however, needs to be proven.
Electrolyte Imbalance
The patient may demonstrate hypokalemia and hyperglycemia in response to stress and trauma. β-Adrenergic receptor stimulation from epinephrine (adrenaline) causes a decrease in serum potassium by driving potassium into the cells. Similarly, when pH is elevated, as is common in the brain-injured patient in whom hyperventilation is used to reduce ICP, potassium is driven into cells as hydrogen ions are released. Decreases in serum potassium values associated with acute hyperventilation and stress do not need to be treated, because total body potassium is unchanged. However, diuretic-induced renal losses of potassium do require replacement to avoid complications of acute intracellular potassium depletion, including potentiation of neuromuscular blockade and cardiac dysrhythmias. Often, in the acutely brain-injured patient, the cause of hypokalemia is multifactorial. Initiation of treatment depends on the predominant clinical circumstances.
Diabetes insipidus may occur in the patient with basilar skull fracture or severe head injury involving the hypothalamus or posterior pituitary. Antidiuretic hormone (ADH) is synthesized in the hypothalamus and secreted by the posterior pituitary. ADH enhances the permeability of free H 2 O in the distal convoluted tubule and collecting duct of the kidney. Patients with diabetes insipidus can lose large volumes (25 L/day) of dilute urine, resulting in marked increases in serum sodium and osmolality.
Diabetes insipidus should be considered in the differential diagnosis of polyuria in any patient with head trauma or pituitary and hypothalamic lesions. The differential diagnosis of intraoperative polyuria includes excessive fluid administration, osmotic agents (eg, hyperglycemia with serum glucose level greater than 180 mg/dL, mannitol), diuretics, paradoxic diuresis in patients with brain tumor, and nephrogenic and central diabetes insipidus. Diabetes insipidus is diagnosed intraoperatively by the ruling out of iatrogenic causes and hyperglycemia, and through a demonstration of marked increases in serum sodium and osmolality with low urine osmolality.
Treatment of diabetes insipidus involves adequate fluid replacement with half-normal (0.45%) saline and administration of ADH. Five percent dextrose in water may alternatively be used; however, caution should be applied to avoid hyperglycemia. Aqueous vasopressin may be given subcutaneously or intramuscularly (5 to 10 U) every 6 hours or as a slow intravenous infusion (up to 0.01 U/kg/h) for rapid control of intraoperative or postoperative diabetes insipidus. Larger doses may cause hypertension. For less frequent dosage, desmopressin (DDAVP) 1 to 4 μg intravenously or subcutaneously every 12 hours may be administered. Desmopressin may be given more frequently if the diabetes insipidus is not controlled. This agent has less vasopressor activity than aqueous vasopressin and is preferable to vasopressin in patients with coronary artery disease and hypertension.
Hyponatremia may also occur in the acutely brain-injured patient. Hyponatremia may be associated with diminished (eg, diuretic usage, adrenal insufficiency, salt-losing nephritis), expanded (eg, congestive heart failure, renal failure), or normal (eg, hypothyroidism, syndrome of inappropriate ADH secretion) extracellular fluid volumes. Rapid reduction of the serum sodium value to less than 125 to 130 mEq/L may cause changes in mental status and seizures. The first step in diagnosis is to establish the category to which the patient belongs. Although many clinicians are quick to suggest syndrome of inappropriate ADH secretion in patients with brain injury, this diagnosis should be made only after exclusion of other possible causes. In neurosurgical patients, hyponatremia is most commonly associated with intravascular volume depletion caused by diuretic administration or a loss of sodium via the kidney. After SAH, patients may have a primary natriuresis (“cerebral salt wasting”), which, unlike syndrome of inappropriate ADH secretion, is associated with a decreased intravascular volume. Aggressive fluid therapy is required in patients with SAH to maintain a normal intravascular volume.
Blood toxicology screen is indicated in patients with impaired neurological status as alcohol, cocaine and other sympathomimetic drugs are associated with both TBI and spontaneous ICH. Pregnancy test should be performed in women of childbearing age.
Management of the Brain-Injured Patient
Airway
Patients with a GCS score of 8 or less or uncooperative patients who require sedation for neuroimaging require intubation, because patients with suspected head injury should not be sedated without an endotracheal tube in place and control of ventilation ensured. Trauma patients should be assumed to have a cervical spine injury until proven otherwise. Nasal intubation should be avoided in patients with suspected basilar skull fractures and sinus injuries.
The patient’s airway and hemodynamic status should be quickly assessed before a plan for endotracheal intubation is chosen ( Fig. 10.4 ). Rapid sequence induction with cricoid pressure is a standard technique for establishing a definitive airway in the neurologically compromised patient. Comatose patients may not require sedation or paralysis for direct laryngoscopy and endotracheal intubation. In trauma patients, in-line stabilization of the neck should be utilized. Newer airway equipment, such as optical and video laryngoscopes (eg, AirTraq, GlideScope®, McGrath®) and stylets (Shikani Seeing Stylet), may be utilized to improve visualization of the glottic opening. However, fiberoptic laryngoscopy is still considered the “gold standard” for management of the difficult airway, especially if concomitant cervical spine injury is suspected. In some cases, a cricothyroidotomy may be required.

If the airway appears normal, a muscle relaxant should be given to facilitate glottic exposure and reduce coughing. The prevention of the cough by the prior administration of a muscle relaxant appears to be most important in preventing significant increases in ICP with endotracheal intubation. Nondepolarizing neuromuscular blockers have been shown to prevent increases in ICP during tracheal stimulation. Additionally, White and colleagues found that succinylcholine, but not thiopental, fentanyl, or lidocaine, prevented marked rises in ICP with endotracheal suctioning in comatose head-injured patients, because of the greater reduction in coughing after administration of succinylcholine. However, barbiturates and lidocaine were effective in attenuating the increase in ICP due to tracheal stimulation in patients who also received a muscle relaxant. , These drugs and narcotics may also be necessary to prevent increases in mean arterial pressure and ICP with endotracheal intubation.
One should assess the patient’s hemodynamic status to choose an anesthesia induction agent that reduces the increase in ICP that accompanies endotracheal intubation. The primary goal is to maintain adequate cerebral perfusion pressure (CPP) by ensuring hemodynamic stability while reducing ICP. Severe hypotension from the inappropriate administration of a large dose of propofol in a hypovolemic patient may be worse for the patient than the transient rise in ICP that accompanies endotracheal intubation. If the patient is hypertensive or hemodynamically stable, a rapid-sequence induction with head and neck stabilization, defasciculation, cricoid pressure, and administration of propofol, lidocaine, and succinylcholine can be used. To avoid hypoxemia and excessive increases in PaCO 2 , the patient may be manually hyperventilated while cricoid pressure is maintained before intubation. If the patient is hypertensive, administration of narcotics (such as fentanyl), antihypertensive agents, or both may be necessary to prevent severe hypertension and increases in ICP with endotracheal intubation, but extreme caution is required to prevent untoward reductions in CPP in this setting. Esmolol and labetalol have less potential to raise ICP than sodium nitroprusside, which is a marked cerebrovasodilator. Calcium channel blockers nicardipine and clivedipine are potent and safe antihypertensive medications which are widely used, particularly in patients with ICH. , If the patient is somewhat hypovolemic (as is common in the patient with multiple injuries), etomidate (0.2–0.4 mg/kg) may be used as an alternative to propofol because it is effective in reducing cerebral blood flow (CBF) and ICP.
There is a renewed interest in ketamine as an induction agent in patients with brain injury. A recent randomized controlled trial proved safety of ketamine (2 mg/kg) while providing similar intubating conditions compared to etomidate in 655 patients with brain injury requiring emergency intubation. If hypertension and tachycardia are of concern, another option would be a mixture of ketamine 0.75 mg/kg and propofol, which provides more stable hemodynamics compared to induction with propofol only. Ketamine (1 mg/kg) was shown to decrease intracranial pressure in adults during anesthesia and recently in ICU children with increased ICP (ketamine 1–1.5 mg/kg).
Although succinylcholine may transiently increase ICP because of greater CO 2 production or cerebral stimulation from the fasciculations, succinylcholine is the muscle relaxant of choice in emergent endotracheal intubation because of its fast onset of action, short duration, and excellent intubating conditions, unless it is contraindicated. Whether pretreatment with a “defasciculating” dose of nondepolarizing muscle relaxant is effective preventing succinylcholine-induced increase in ICP is not clear. The benefits of rapid intubation and hyperventilation, if required, outweigh the disadvantages of brisk ICP increase during intubation in the acutely injured patient. Succinylcholine can potentially cause hyperkalemia. Rocuronium (0.6–1.2 mg/kg) should be chosen in this circumstance, assuming that the airway is not difficult.
Goals in the Acute Care of the Brain-Injured Patient
The principal aim in the acute medical management of the brain-injured patient is to prevent secondary neurologic injury. Over the last 30 years, an understanding of the pathophysiology and goals of an early treatment of the potential causes of secondary neurologic injury led to a significant decrease in mortality of patients with severe TBI, from 50% to 25–30%.
Secondary brain injury is an important determinant of outcome from severe TBI. Factors contributing to the development of secondary neurologic injury include hypoxia, hypercapnia, hypotension, intracranial hypertension, and transtentorial or cerebellar herniation. Durations of systemic hypotension with systolic blood pressure (SBP) 90 mmHg or lower, hypoxia with arterial O 2 saturation (SaO 2 ) 90% or lower, and pyrexia with core temperature 38° C or higher have been found to be strongly associated with mortality after TBI. Many of these factors are potentially treatable. Both CPP greater than 90 mmHg and higher GCS scores correlated with better neurologic outcome, whereas CPP lower than 50 mmHg is independently associated with poor outcome after TBI. Box 10.4 summarizes the aims in the acute care of patients with TBI injuries.
- A.
Prevention of hypoxemia—maintain PaO 2 > 60 mmHg or SaO 2 > 90%:
- 1.
Increase inspired oxygen tension.
- 2.
Treat pulmonary pathologic condition.
- 3.
Consider positive end-expiratory pressure (10 cm H 2 O or less).
- 1.
- B.
Maintenance of blood pressure:
- 1.
Prevent hypotension—maintain systolic blood pressure (SBP) > 90 mmHg:
- a.
Avoid glucose-containing solutions.
- b.
Maintain intravascular volume status—aim for euvolemia.
- a.
- 2.
Treat hypertension:
- a.
Sympathetic nervous system overactivity.
- b.
Increased intracranial pressure.
- c.
Light anesthesia.
- a.
- 1.
- C.
Reduction in intracranial pressure:
- 1.
Head position.
- 2.
Brief periods of hyperventilation.
- 3.
Hyperosmolar therapy.
- 4.
Sedation.
- 5.
Hypothermia.
- 6.
Surgical procedures: drainage of cerebrospinal fluid and evacuation of hematoma.
- 1.
High-dose steroids do not reduce ICP in TBI and do not affect outcome from severe head injury. They are therefore not recommended for the treatment of acute TBI. Steroids may be useful in reducing edema in the rare patient who presents with impending brain herniation from a brain tumor. In such a patient, clinical improvement occurs within hours of the initiation of steroid therapy.
Prevention of Hypoxemia
Prompt and aggressive treatment of hypoxemia in TBI patients is imperative because hypoxemia is associated with the development of secondary brain injury, worsening neurologic outcome, and increasing mortality, especially when associated with systemic hypotension. According to a study of 717 cases in the Traumatic Coma Data Bank, hypoxemia was identified in 22.4% patients with severe TBI and was associated with increases in morbidity and mortality.
Every patient with brain injury should be assessed according to general “ABC” principles of trauma management (airway, breathing, circulation), which is initiated with provision of an adequate airway and breathing/ventilation. Every brain-injured patient should receive supplemental oxygen regardless of initial GCS score. Oxygenation should be monitored whenever possible with pulse oximeter or by measurement of arterial blood gas levels. The minimum goal of oxygenation in patients with brain injury should be to maintain SaO 2 at 90% or higher or PaO 2 at 60 mmHg or higher. Patients with severe brain injury should be intubated and ventilated with 100% oxygen until adequate oxygenation is verified.
The possibility has been raised that positive end-expiratory pressure (PEEP) may increase ICP in the brain-injured patient because it may increase cerebral venous volume by reducing cerebral venous outflow. However, 10 cm H 2 O PEEP improves oxygenation and usually results in clinically inconsequential increases in ICP in patients with severe head trauma. PEEP may affect ICP less in patients with the stiffest lungs, who are presumably the ones who need PEEP the most.
Maintenance of Hemodynamic Stability
Hypotension
When systemic hypotension is defined as SBP less than 90 mmHg, even a single recorded prehospital episode has been shown to correlate with higher morbidity and mortality in patients with TBI. While patients are in the intensive care unit, the duration of hypotension (SBP < 90 mmHg) strongly correlates with worsening of neurologic outcome as assessed by the GCS and with an increase in mortality. Blood pressure should be monitored and SBP should be kept higher than 90 mmHg.
The most common hemodynamic problem in the patient with head trauma and multiple injuries is hypovolemia caused by blood loss, profound diuresis from mannitol, and inappropriate attempts to restrict fluid intake. Because the damaged brain tolerates hypotension poorly, intravenous fluids should be administered in sufficient quantities to rapidly restore intravascular volume and CBF. Intraoperative blood loss may be severe in vascular injuries and skull fractures. Massive volume replacement may be required intraoperatively if a dural sinus is injured. In addition, blood loss may be difficult to quantify because it spills on the drapes and on the floor. Patients with large intracranial hemorrhages, with normal blood pressures in the lower range (SBP 100–120 mmHg), or with relative tachycardia (heartbeat > 100 beats/min), should be considered to be hypovolemic unless proven otherwise. The hypovolemia may manifest as severe hypotension when the brain is decompressed. With the acute reduction of ICP, sympathetic tone and systemic vascular resistance are diminished, unmasking profound intravascular volume depletion. Vasopressors may be helpful in restoring blood pressure while fluid is being given to restore intravascular volume. However, overzealous fluid administration that elevates cerebral venous pressure may exacerbate brain edema.
The presence of hypovolemia is best assessed from clinical signs such as hypotension, tachycardia, an inability to tolerate anesthetic agents, and SBP variations with positive-pressure ventilation. A drop in SBP greater than 10 mmHg with positive-pressure ventilation is a sensitive indicator of a 10% reduction in blood volume ( Fig. 10.5 ). This decrease in SBP is a significantly better indicator than the central venous pressure.

The variation in SBP correlates well with the degree of hemorrhage in dogs and humans. However, placement of a central venous line may be helpful in the acute setting, especially to prevent overhydration, and should ultimately be performed to help guide fluid replacement. Timely evacuation of the brain mass must be accomplished first, with placement of central line later, after surgery has begun and the patient has been stabilized.
Hypertension
Patients are often hypertensive after an isolated brain injury, because of an increase in catecholamines from stress-induced activation of the sympathetic nervous system. Because autoregulation may be impaired after head trauma, hypertension may cause brain hyperemia, promote the development of vasogenic edema, and further increase ICP. However, before immediate treatment of the blood pressure elevation with an antihypertensive agent, other causes of hypertension (such as increased ICP and inadequate anesthesia) should be first eliminated. Ensuring adequate oxygenation and ventilation, placing the head up slightly in neutral position, preventing coughing, or administering propofol alone or together with narcotics may help reduce ICP and provide greater anesthesia. Usually these measures are effective to control blood pressure in the acute management of the brain-injured patient. However, in the patient with preexisting hypertension or the severely hyperdynamic patient, control of hypertension with β-adrenergic antagonists (eg, metoprolol, labetalol, esmolol) may also be indicated. These drugs specifically treat the cause of hypertension (eg, sympathetic overactivity), and they are not cerebral vasodilators. Prophylactic β-adrenergic receptor blockade may also be useful in reducing supraventricular tachycardia, ST-segment and T-wave changes, and myocardial necrosis associated with severe head injury. Calcium channel blockers such as nicardipine and clevidipine are also suitable to control hypertension. Systemic vasodilators, such as sodium nitroprusside, nitroglycerin, and hydralazine, may increase ICP and should be avoided. , ,
In nontrauma patients, vomiting, hypertension of systolic blood pressure > 200 mmHg, along with severe headache and fast progression of neurological deficit, are highly suspicious for ICH. Arterial hypertension in patients with ICH contributes to enlargement of hematoma, rebleeding and brain edema. Rapid diagnostic neuroimaging and aggressive gradual control of blood pressure with continuous intravenous antihypertensive medication is required, while maintaining cerebral perfusion pressure not less than 60 mmHg. Recently, a rapid, within 1 hour, decrease in blood pressure lower than 140 mmHg was shown to be safe; however, whether it is beneficial to outcome is not clear yet. There is currently an on-going multicenter study which might clarify this question ( http://atach2.com/ ). Calcium channel blockers nicardipine and clivedipine, along with alpha- and beta-blockers and other medications, can be safely used for blood pressure control utilized.
Fluid Management (see Chapter 9 )
Iso-osmolar crystalloid solutions should be used to replace intravascular volume. These include Plasma-Lyte, Normosol-R, and 0.9% normal saline. Although use of any of these solutions may considerably raise ICP after resuscitation from shock, ICP would be higher after inadequate resuscitation because of the development of cytotoxic edema from cerebral ischemia. The blood–brain barrier may be damaged by head trauma, allowing all fluids to cross the blood–brain barrier. Overhydration, causing increases in central venous pressure, should be avoided. Therefore, the aim of fluid management is to maintain a euvolemic patient. Less urgent procedures (eg, orthopedic surgeries) that entail significant blood loss should be delayed as long as the brain injury has stabilized (eg, ICP is within normal limits). Lactated Ringer’s solution should be avoided because of its hypo-osmolarity.
The choice between colloid and crystalloid solutions for fluid replacement remains controversial. Water movement across the blood–brain barrier depends primarily on the difference in osmolality between plasma and brain. Decreases in oncotic pressure may not affect brain water content much because oncotic pressure makes only a small contribution to total plasma osmolality. Most experimental studies suggest that brain water content and ICP are no different whether isotonic colloid or crystalloid solutions are administered. Although there is experimental evidence that colloids may result in less brain edema in a model of head trauma, this finding has not been supported by human studies. Investigators of the SAFE (Saline versus Albumin Fluid Evaluation) trial analyzed results from a subgroup of 460 patients with TBI who were randomized to receive albumin or 0.9% saline for the initial fluid resuscitation. The authors demonstrated a strong association of albumin administration with poor neurologic outcome (52.7% in albumin versus 39.4% in saline group) and death (33.2% in albumin versus 20.4% in saline group) at 24 months after TBI. Significantly higher morbidity and mortality with albumin than with saline was found for patients with severe TBI, with GCS score 3 to 8 at admission, but not with moderate TBI. The mechanism of such a dramatic influence of albumin on the outcome after TBI is not clear; however, aggravation of brain edema following movement of albumin across the disrupted blood–brain barrier has been hypothesized. This theory is also supported by another randomized clinical trial, in which 90 patients with TBI were randomly assigned to receive initial fluid resuscitation with either fresh frozen plasma or 0.9% saline, which demonstrated a higher incidence of intracerebral hematoma formation and an increased 1-month mortality after the use of fresh frozen plasma.
Hydroxyethyl starch (HES) was once the most commonly administered colloid in the treatment of critically ill patients. However, practitioners are now shying away from the use of HES due to its association with severe complications, including coagulopathy and renal failure. HES prolongs the partial thromboplastin time (PTT) through a decrease in factor VIII coagulant activity and von Willebrand factor level, and volumes in excess of 500 mL may put the patient at increased risk for an intracranial hematoma. Several studies have examined the use of 6% HES in critically ill patients and found a significant increase in the incidence of kidney injury, the need for renal replacement therapy, and the incidence of adverse events compared to 0.9% saline. , The impact on mortality is less clear. The European Society of Intensive Care Medicine released a consensus statement in 2012 recommending against the use of HES in organ donors and in patients with severe sepsis, head injury, or at risk for acute kidney injury. In 2013, the United States Food and Drug Administration recommended that HES solutions not be used in critically ill adult patients and mandated that a boxed warning regarding the increased risk of mortality, severe renal injury, and excessive bleeding be included in the package insert for HES products. Based on this evidence, it is prudent to avoid the use of HES in the management of patients with acute brain injury.
Hypertonic saline (HS) solutions have a potential advantage over other solutions in the resuscitation of hypotensive brain-injured patients because of their ability to reduce brain water content and ICP , and to stabilize blood pressure. Small-volume (bolus of 250 mL) resuscitation with 7.5% HS in hypotensive patients with multiple trauma and TBI has been shown to be safe and tends to better stabilize blood pressure and to improve in-hospital survival in patients with severe TBI (GCS score < 8) than lactated Ringer’s solution. , However, when the same regimens of fluid resuscitation were compared in patients with GCS scores less than 9 and SBP lower than 100 mmHg, the neurologic outcome at 6 months after injury in patients who received HS did not differ from that in the patients who received lactated Ringer’s solution.
There are no current data about an optimal concentration and volume of HS that can be recommended for fluid resuscitation. However, with safety and potential benefits taken into account, use of HS for fluid resuscitation in a hemodynamically unstable patient with brain injury represents one of the reasonable options. Other concentrations of HS—1.6% or 3% —may be used as well. Because the osmolality values of 3% HS and 20% mannitol are close, 5 mL/kg of 3% HS will provide the same osmolar load as 1 g/kg of 20% mannitol. To date, there are very few clinical situations in which the use of HS is of major concern. Although rapid correction of sodium in a patient with chronic hyponatremia may potentially predispose to the development of central pontine myelinolysis, the latest literature does not reveal any cases of central pontine myelinolysis after resuscitation with HS, even after repeated boluses. On the other hand, continuous administration of HS in patients with a syndrome of inappropriate ADH secretion may potentially aggravate hyponatremia owing to accumulation of fluid, so HS should not be used as a first-line drug; it should be given only after water restriction and a diuretic regimen have been initiated.
In summary, iso-osmotic solutions (eg, Plasma-Lyte and 0.9% saline) are recommended for initial fluid resuscitation after brain injury. Choice of fluid should be adjusted to levels of blood electrolytes. Prophylactic administration of fresh frozen plasma is not recommended and should be used judiciously in patients with signs of coagulopathy.
Blood Glucose Control
In critically ill neurologic patients stress-induced hyperglycemia is common, and has been strongly associated with increased morbidity and mortality after stroke, , SAH, TBI, , and cardiac arrest. In these human studies, whether the hyperglycemia was the cause or the result of the increased severity of the neurologic damage is unclear.
Although cellular mechanisms of hyperglycemia, such as an impaired immunity and an increased risk of infection, mitochondrial damage, intracellular acidosis, endothelial injury, and inflammation, have been described, the exact mechanisms for the enhanced neurologic damage are unclear. How glucose administration affects outcome after brain injury also is not known. In a rat model of head trauma, glucose administration did not alter neurologic outcome or the formation of brain edema.
The exact threshold level of blood glucose value above which ischemic neurologic damage is increased is also not known; however, it appears to be less than 200 mg/dL. On the other hand, tight glycemic control (maintenance of a blood glucose level at 80–100 mg/dL) using intensive insulin therapy, which has been broadly advocated in the surgical critically ill population, has been recently shown to be detrimental. Tight glycemic control may be particularly dangerous in patients who had experienced some degree of brain injury, such as after cardiac arrest or SAH, because of high incidence of hypoglycemia, lack of benefit, or worsening of outcome. Glucose is the main energy source of the brain, and due to its limited capacity for glycogen storage, the brain has limited compensatory capacity against hypoglycemia. Additionally, the acutely injured brain has a higher metabolic demand than under physiologic conditions and is thus prone to glucose shortage.
Glucose-containing solutions should not be administered acutely to the brain-injured patient because they may exacerbate neurologic damage. Numerous animal studies provide convincing evidence that glucose administration, with or without marked hyperglycemia, enlarges infarct size and augments neurologic damage from global and regional cerebral ischemia.
Part of the difficulty in determining a “safe” level of blood glucose in patients is that blood glucose levels may not accurately reflect brain glucose levels during periods of transient hyperglycemia. Brain glucose values remain elevated after glucose infusion, whereas blood glucose values decrease in response to insulin. Meta-analysis of 16 randomized controlled studies (1248 patients) of the last decade demonstrated that, although tight glycemic control of blood glucose between 70 and 140 mg/dL had no impact on mortality in patients with an acute neurologic injury (TBI, stroke, CNS infections and SCI) compared to conventional glycemic control maintaining blood glucose between 144 and 300 mg/dL, it worsened neurological outcome. These data suggest that maintaining blood glucose between 140 and 180 mg/dL would be an appropriate strategy of glycemic control in patients with acute CNS injury. However, the actual benefits and risks of acute reductions of blood glucose in patients with acute and chronic hyperglycemia are unclear.
Temperature Control
Hyperthermia (temperature > 38° C) is known to be detrimental for patients with brain injury and is strongly associated with worsening neurologic outcome and increasing mortality in patients with TBI, SAH, and stroke. Undoubtedly, hyperpyrexia in patients with brain injury should be avoided and treated. Aggressive warming up of patients with traumatic brain injury who were hypothermic at arrival has been shown to be detrimental; therefore, fast infusion of warmed fluids should be performed cautiously, especially in the operating room setting in cases of hypovolemia and hemorrhage, with the danger of hyperpyrexia in mind.
Treatment options to reduce temperature in patients with acute brain injury include (1) antipyretic medications (aspirin, acetaminophen, ibuprofen, diclofenac), which can be used in patients with stroke but not in those with TBI because of potential worsening of coagulation, (2) external devices such as cooling blankets, which are the most safe and useful tools, and (3) internal cooling such as an intravenous infusion of cold saline and, in resistant hyperpyrexia, endovascular cooling. Currently, there are no data available demonstrating the optimal treatment of the febrile patient with acute neurologic injury, so the treatment approach should be specifically chosen for each patient.
From the physiologic point of view, induction of hypothermia in the patient with brain injury offers the potential benefit of reducing the cerebral metabolic rate of oxygen. Currently there are not sufficient data for a strong recommendation of inducing mild hypothermia in brain-injured patients. Based on the analysis of six randomized control trials performed during the last 15 years (target cooling temperature between 32° and 35° C), Brain Trauma Foundation 2007 guidelines suggested that moderate hypothermia may potentially improve outcome after TBI, suggesting that initiation of hypothermia targeted to 32° to 33° C should be induced and maintained for at least 48 hours after injury to decrease the mortality rate. However, concomitant use of barbiturates and hypothermia can significantly raise the incidence of pneumonia, and, therefore, in patients with increased ICP, the conventional treatment including barbiturates should be applied first, before hypothermia is considered.
Monitoring and Treatment of Intracranial Hypertension
A major goal in the acute treatment of the brain-injured patient is to reduce ICP, which may be accomplished through head position, hyperventilation, hyperosmotic solutions and diuretics, barbiturates and other IV anesthetics such as propofol, and surgical treatment. Monitoring of ICP, CPP, oxygen saturation in the jugular bulb (SjO 2 ), and brain tissue oxygen (PbO 2 ) are useful tools for the bedside assessment of patients with brain injury.
Head Position
A slightly head-up position (up to 30-degree head-up tilt) with the neck in neutral position promotes cerebral venous drainage and reduces ICP if the cerebrospinal fluid pathways are still patent. Lateral turning of the head, tight endotracheal or tracheostomy tube ties around the neck, and the Trendelenburg position may dramatically raise ICP because they restrict venous return from the brain. The patient’s respiratory muscles should be paralyzed to prevent coughing and bucking on the endotracheal tube, which also increases ICP. On the other hand, marked elevation of the head in a hypovolemic patient, if it diminishes mean arterial pressure, may cause decreased brain perfusion and cerebral ischemia.
Hyperventilation
Hyperventilation-induced hypocapnia is a powerful therapeutic tool in treatment of intracranial hypertension. Although hypocapnia may acutely lower ICP by causing an extracellular alkalosis and cerebral vasoconstriction, it may increase cerebral ischemia. Excessive hyperventilation causes profound cerebral vasoconstriction and a decrease in CBF, which may be detrimental if CBF was already impaired as a result of brain injury. Taking into account that a majority of brain-injured patients suffer a dramatic decrease in CBF to less than 50% of normal in the first 24 to 48 hours after injury, , the Brain Trauma Foundation’s 2007 guidelines recommend avoiding hyperventilation in the first 24 hours after brain injury and avoiding prophylactic hyperventilation.
A randomized, controlled trial of prophylactic hyperventilation therapy for 5 days after severe TBI also found that outcome was worse in the hyperventilated group, but only in patients with relatively intact motor function. This result may reflect the ability of hypocapnic vasoconstriction to exacerbate cerebral ischemia in the injured brain. Global CBF and regional CBF are severely reduced and metabolism is increased during the first few hours and days after brain injury. Focal cerebral ischemia is common after TBI. Hyperventilation causes a further drop in CBF, often without a decrease in ICP, which may further exacerbate cerebral ischemia.
Hyperventilation may have significant adverse cardiopulmonary effects. It may lower systemic blood pressure by reducing venous return, sympathetic stimulation, and cardiac output. It results in a leftward shift of the oxyhemoglobin dissociation curve, causing lower mixed venous and arterial oxygen tension values for any given oxygen saturation. Hypocapnia inhibits hypoxic pulmonary vasoconstriction and causes bronchoconstriction. Increases in pulmonary shunt may occur in hyperventilated patients.
Because of these concerns, PaCO 2 generally should be kept at normocapnic levels in the patient with TBI. Brief periods of hypocapnia may be useful and have not been shown to be harmful in the acutely decompensating patient until definitive surgical treatment takes place. According to the Brain Injury Foundation’s guidelines for the management of severe traumatic brain injury, if hyperventilation is considered for treatment, a jugular venous bulb catheter should be inserted for monitoring of SjO 2 . Jugular desaturation is common after head injury and traumatic intracranial hematoma, and it may be exacerbated by hyperventilation. High interpersonal variability in normal levels of SjO 2 (55–75%) may make interpretation of the parameter difficult in the brain-injured patient. However, the majority of experts believe that a decrease in SjO 2 to less than 50% reflects a decrease in CBF, which could potentially lead to brain ischemia and should be avoided.
Hyperosmolar Therapy
Mannitol decreases brain water content and ICP primarily by increasing plasma osmolality, thereby creating an osmotic gradient across the intact blood–brain barrier. The amount of water that can be withdrawn from the brain depends on the magnitude of the osmotic gradient, the total time the gradient exists, and the integrity of the blood–brain barrier. Mannitol is less effective with larger lesions because with damaged blood–brain barriers, mannitol moves down its concentration gradient into the brain. This movement may account for a rebound increase in ICP occasionally seen after mannitol infusion.
Administration of mannitol may cause a triphasic hemodynamic response. Transient (1 to 2 minutes) hypotension may occur after rapid administration of mannitol. Mannitol then increases blood volume, cardiac index, and pulmonary capillary wedge pressure, with a maximum increase shortly after termination of the infusion. ICP may rise transiently because of increases in cerebral blood volume and CBF. ICP increases are attenuated by slow infusion of mannitol. Transient rises in ICP are uncommon in the patient with elevated ICP. At 30 minutes after mannitol administration, blood volume returns to normal, and pulmonary capillary wedge pressure and cardiac index drop to below normal levels because of peripheral vascular pooling.
Mannitol reduces blood viscosity and red blood cell rigidity, which may enhance perfusion of the brain microcirculation. This agent transiently reduces hematocrit and increases serum osmolality. It also causes hyponatremia, hyperkalemia, and decreases in pH caused by HCO 3 dilution. Prolonged and marked hyperosmolality with hyponatremia can occur in patients with acute or chronic renal failure.
Doses of mannitol from 0.25 to 2 g/kg are usually administered; a typical dose is 1 g/kg. Lower doses are effective in reducing ICP acutely and cause fewer electrolyte abnormalities; however, they must be given more frequently. Rapid administration of mannitol causes a more profound reduction in ICP but may transiently cause hypotension and more marked increases in intravascular and cerebral blood volumes. The benefits and disadvantages of a particular dose and speed of administration must be weighed carefully in any patient. Giving more mannitol when the patient’s serum osmolality value is higher than 330 mOsm/L is seldom effective.
Mechanisms of action of hypertonic saline on the cerebral physiology resemble those of mannitol. Equiosmolar boluses of HS and mannitol have been shown to have similar effects on brain shrinkage in patients undergoing craniotomy with and without SAH as well as on the decrease in ICP in patients with TBI. However, HS does not cause as profound a diuresis or as negative a fluid balance as mannitol does. Therefore, administration of HS can be beneficial and should be considered in hemodynamically unstable or hypovolemic patients or patients with heart disease. Boluses of HS may be administered intravenously over 15 to 20 minutes. No exact bolus volume or concentration of HS solution can be strongly recommended. A wide range of HS solution concentrations, between 1.6% and 7.5%, have been successfully administered peripherally without significant complications. Administration of 5 mL/kg of 3% HS will provide the same osmolar load as 1 g/kg of 20% mannitol.
Diuretics
Furosemide has been reported to lower ICP and brain water content when used alone in large (1 mg/kg) doses or in combination with mannitol in smaller doses. In contrast to mannitol, furosemide alone did not reduce ICP. Clinical impressions are that mannitol produces a better reduction of brain bulk than furosemide. Furosemide may be advantageous to patients with heart and renal diseases because, unlike mannitol, it does not increase blood volume or ICP.
The mechanism of furosemide’s action on reducing ICP is unknown. It is not related to the agent’s diuretic effect. Furosemide may reduce cerebrospinal fluid formation and water and ion penetration across the blood–brain barrier. It also potentiates mannitol by sustaining the increase in serum osmolality induced by mannitol. Therefore, reductions in ICP and brain shrinkage are consistently greater and longer in duration with mannitol and furosemide than with either agent alone. However, hyponatremia, hypokalemia, hypochloremia, hyperosmolality, and a significantly greater rate of water and electrolyte excretion occur with this combination of diuretics. Water excretions of up to 42 mL/min have been reported with the combination of drugs, compared with 17 mL/min with mannitol alone.
Low doses of furosemide (5–20 mg) added to mannitol (0.25–1 g/kg) are very effective in reducing brain bulk. Larger doses of furosemide may be required to produce the same effect in the patient who had been undergoing long-term diuretic therapy. Mannitol-induced increases in blood volume and ICP may also be attenuated when furosemide is administered before mannitol. However, with administration of combined diuretics, vigorous intravascular fluid and electrolyte replacement are required. A urine loss of 2–3 L over 2 hours is common with combined diuretic therapy.
Barbiturates and Other Sedatives and Analgesics
Barbiturates reduce cerebral metabolism, CBF, cerebral blood volume, and ICP. Due to a lack of availability of short-acting barbiturates in the United States, barbiturate administration is infrequent and primarily reserved for treatment in the intensive care unit. High-dose barbiturates have been used successfully to reduce ICP in patients with TBI refractory to other treatments and are considered the gold standard for sedation in patients with refractory intracranial hypertension. However, barbiturate therapy probably does not affect long-term outcome after TBI.
Although barbiturates may aid in the acute control of ICP, their use is limited by the fact that they may cause cardiac depression as well as reduce arterial blood pressure and CPP. Because hypotension is a definite risk factor for worsened neurologic outcome in the brain-injured patient, maintaining a normal blood pressure is particularly important. Thus barbiturates should be carefully titrated, and blood pressure supported with vasopressors or inotropic agents as necessary. Barbiturates should not be administered to hypovolemic or hypotensive patients. High doses of barbiturates should be administered with caution before the surgical evacuation of an intracranial mass, because blood pressure may decrease with the reduction in sympathetic tone that accompanies brain decompression. Pentobarbital is the most useful barbiturate for the control of ICP. The classic regimen, which was recommended for inducing barbiturate coma, consisted of a loading dose of pentobarbital, 10 mg/kg over 30 minutes, followed by 5 mg/kg/hour for 3 hours, followed by a maintenance infusion of 1 mg/kg/hour. However, other regimens may be successfully used, especially if burst suppression is controlled by EEG. Given the instability of the acutely injured patient, high doses of barbiturates are best reserved for use in the intensive care unit.
Propofol is useful for sedation and control of ICP. Like barbiturates, propofol may cause a decrease in blood pressure; however, the major advantage of propofol is the fast recovery from its effect, which provides favorable conditions for neurologic examination. The dose of propofol required for the burst suppression is high (about 200 μg/kg/min) and therefore provides a high lipid load to the patient. Long-term high-dose (> 5 mg/kg/hour for > 48 hours) propofol sedation has the potential for the development of the fatal propofol-infusion syndrome. This condition is characterized by lactic acidosis, rhabdomyolysis, renal failure, lipidemia, fatty infiltration of liver, and fatal cardiac failure. Propofol infusion syndrome has been more commonly reported in children, and its use is not advised for long-term infusion for children in the intensive care unit. It has been shown to have a strong association with neurologic disease, particularly TBI. The onset of propofol-infusion syndrome may be short—less than 24 hours—so when a patient is in the intensive care unit, the choice of sedative agent should be reconsidered daily.
Midazolam reduces cerebral metabolism, blood flow and blood volume, but preserves cerebral autoregulation and can be successfully used for sedation. Usually, continuous infusion of midazolam (2–4 mg/hour) is combined with opioids (morphine sulfate 4 mg/hour, fentanyl 2–5 μg/kg/h, or sufentanil 0.05–2 μg/kg/h). Because opioids generally do not affect cerebral metabolism and blood flow and do provide hemodynamic stability, these regimens are well tolerated. However, slow recovery after cessation of infusion makes midazolam-opioid sedation less desirable for patients with brain injury. Reversal of benzodiazepine effects with flumazenil can lead to increases in ICP and seizure activity.
Surgical Treatment
Surgical treatment may be required to lower ICP acutely. Placement of a ventriculostomy catheter may be difficult because the extent of brain swelling may prevent localization of the ventricles.
Prompt removal of an acute subdural, epidural, or large solitary intracerebral hematoma is indicated. Mortality and morbidity are reduced by prompt diagnosis and surgical treatment of an epidural hematoma, especially if performed before signs of tentorial herniation occur. In addition, mortality from an acute subdural hematoma is reduced by rapid diagnosis and surgical treatment. Seelig and colleagues reported that patients who underwent surgery within 4 hours of injury had a 30% mortality rate, compared with 90% mortality for those who had surgery after 4 hours.
Spinal cord injury
Spinal injuries occur in 5–10% of major trauma cases. The National Emergency X-Radiography Utilization Study (NEXUS) group, which prospectively enrolled 34,069 patients with blunt trauma who underwent cervical spine radiography at admission in 21 institutions, found an incidence of cervical spine injuries of 2.4%. Risk factors for cervical spine injury included (1) male gender, (2) age older than 65 years, and (3) ethnicity (white, and “other” ethnicity, non-Hispanic, non-black). Motor vehicle accidents are the most common cause of injury, followed by falls, sports injuries, and gunshot or stab wounds. People experiencing head-first falls and unrestrained (by seatbelts) drivers or passengers in high-speed, front-end motor vehicle accidents are at particularly high risk (6– 10%) for cervical spine injury. At moderate risk (1–3%) are drivers or passengers (unrestrained by seatbelts) in lower-speed motor vehicle accidents and people with blunt head trauma or with side-first or foot-first falls. Totally alert patients without any neck pain or tenderness are generally not at risk for spinal injury, because a significant association is found between neck pain or tenderness and cervical injury. However, if a patient has even minimal spinal tenderness, has other painful injuries, or is intoxicated, the cervical spine should be considered unstable and should be fully evaluated.
Only about 5% of all spinal cord injuries are observed in children. In childhood, fractures are less common because spine mobility is greater than that in adulthood as a result of ligamentous laxity and incompletely ossified wedge-shaped vertebrae. However, these anatomic features make children prone to extremely high cervical lesions and increase the incidence of SCI without radiographic abnormality.
Neurologic Evaluation
Evaluation of the Extent of Spinal Cord Injury
A convenient way to visualize the structure of the vertebral column uses the three-column concept. The anterior column contains the anterior longitudinal ligament and the anterior two-thirds of the vertebral body and annulus fibrosus. The middle column contains the posterior one-third of the vertebral body, annulus fibrosus, and posterior longitudinal ligament. The posterior column consists of the posterior neural arch, spinous processes, articular facet processes, and their corresponding posterior ligamentous column. The three-column concept is useful in localizing spinal injury, depending on the mechanism of injury.
Injuries to the spine may be classified as extension, flexion, compression, rotation, or some combination of these four basic mechanisms ( Fig. 10.6 ). Extension injuries, such as those from blows under the chin or whiplash, mostly disrupt the posterior column. Flexion injuries, such as a diving injury, mostly disrupt the anterior column. The stability of the injured spine is variable and ranges from stable (eg, burst fracture or wedge of a vertebral body) to very unstable (eg, hangman’s fracture). The primary factor that determines the stability of the injury is the integrity of the ligaments, intervertebral disks, and osseous articulators. In addition, the spinal cord may or may not be injured. With a complete SCI, there is loss of all motor or sensory function below the level of the injury. With incomplete injuries, there is some preservation of function. Incomplete lesions may result in several syndromes ( Table 10.1 ). Of patients with significant SCI, 30–70% have neurologic deficits. About 70% of cervical spine injuries are considered potentially unstable or to be associated with clinically significant SCI. Vertebrae C5 to C7 constitute the most vulnerable segment of cervical spine, and fractures, dislocations, or bony injuries in this segment are most likely to result in SCI. However, the degree of SCI cannot be correlated with the stability of the spine.
Syndrome | Signs |
---|---|
Complete neurologic injury | Loss of all motor and sensory function below the level of injury |
Incomplete neurologic injury: | |
Central cord | Motor loss (arms greater than legs) |
Bladder dysfunction | |
Variable sensory loss | |
Brown-Séquard syndrome | Ipsilateral paralysis |
Ipsilateral loss of proprioception, touch, and vibration | |
Contralateral loss of pain and temperature | |
Anterior cord syndrome | Bilateral motor loss |
Bilateral loss of pain and temperature | |
Preservation of proprioception, touch, and vibration | |
Posterior cord syndrome | Loss of touch and temperature |
Motor function intact | |
Proprioception and vibration intact |
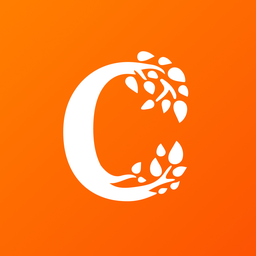
Full access? Get Clinical Tree
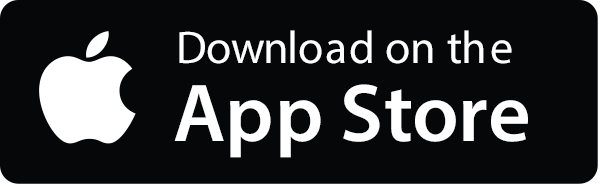
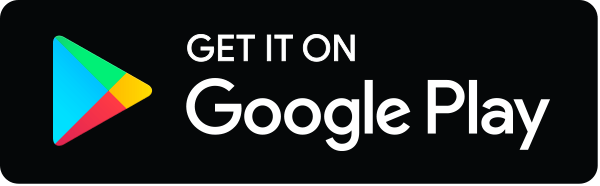
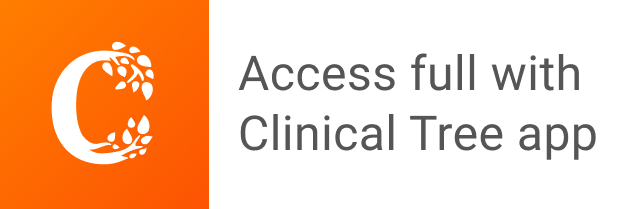