Chapter 30 Cardiac Bypass for Repair of Congenital Heart Disease in Infants and Children
The history of surgery for congenital heart disease is relatively brief. The first successful intracardiac operation took place less than 60 years ago. The groundwork for the performance of cardiac surgery began in two very different arenas. Clinically, surgeons had gained valuable experience in the battle theaters of World War II with removal of foreign bodies from inside the heart.1 Experimentally, DeBakey devised a hand-driven pump in 1934. Later, rudimentary bubble or film oxygenators were added to this device. Another important line of experimentation was pursued by Bigelow and colleagues, who described the application of hypothermia to cardiac surgery in 1950. Although two groups successfully performed surgery to repair atrial septal defects, these were performed without the assistance of cardiopulmonary bypass. F. John Lewis successfully closed an atrial septal defect under deep hypothermic arrest in 19512 and a group led by Gross and Wilkins described the atrial well technique in 1953, whereby they opened the right atrium and sewed to it a silo, which then filled with blood, enabling them to operate on the beating heart by feel.3 Between 1951 and 1954, other groups described various forms of cardiopulmonary bypass. Collectively, there were 18 described operations with only one survivor (operated on May 18, 1953, by J.H. Gibbon). Interestingly enough, the survivor was the first in his series after which he recorded five consecutive deaths, resulting in his abandonment of the technique.4 Failures were attributable to incorrect preoperative diagnoses, ventricular dysfunction at termination of bypass, hypovolemia, surgical errors, and ventricular failure within the convalescence period. During this same period, C. Walton Lillehei and colleagues described a technique known as controlled cross-circulation whereby a “donor,” usually one of the patient’s parents, functioned as a pump/oxygenator (Figure 30-1). The first case was described in 1954. After this, they described 45 patients, with 18 patient and no donor deaths. Although clearly an extremely high mortality, this represented a great improvement.5 Interestingly enough, after this data was published, some commentators suggested abandonment of further research into the bypass machine. In May 1955, a team lead by Kirklin of the Mayo Clinic published an initial series of 8 patients who were operated on by a machine similar to that of Gibbon. Despite a mortality rate of 50%, this was clearly an improvement over the early bypass patients. In conclusion, Kirklin declared that “Use of this system established excellent conditions for precise, unhurried intracardiac surgery.”6 Thus began the modern age of cardiac surgery. What followed over the next few decades was experimentation with different types of oxygenators, culminating in the formulation of microporous hollow-fiber membranes. These oxygenators were made of hollow fibers within a hard plastic shell. They had a large surface area/volume ratio, thus making them extremely efficient for gas exchange. Other advantages included being easy to produce in large numbers, in different sizes, and with noncompliant blood/gas compartments. These collective advantages made them ideal for pediatric cardiac surgery.
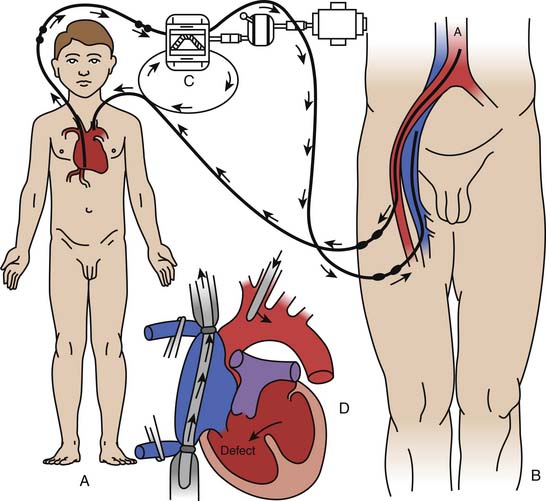
Figure 30–1 Controlled cross circulation.
(Modified from Lillehei CW: Controlled cross circulation for direct-vision intracardiac surgery: correction of ventricular septal defects, atrioventricularis communis, and tetralogy of Fallot, Post Grad Med [Minneapolis] 17:288-396, 1955.)
Cardiopulmonary Bypass in Infants Versus Adults
The management of CPB in the pediatric patient is very different from that of the adult (Table 30-1). The most important of these are the routine use of hypothermia with or without circulatory arrest, the use of low perfusion pressures, and, of great clinical importance, marked hemodilution. Thus pediatric patients are exposed to the extremes of physiologic tolerance. These factors, coupled with immature organ systems, place pediatric patients at great risk during the period of cardiopulmonary bypass. In addition to these differences, which are directly attributable to the differences between the two age groups, there are additional factors unique to the pediatric patient that further complicate the management strategy. Box 30-1 lists some of these factors. From the surgical perspective, several technical challenges exist. Venous and arterial cannulae have potential effect on hemodynamics. Collateral vessels may allow blood return to the heart during bypass (making visibility a challenge). Collaterals may also steal blood away from vital organs making organ preservation difficult.7 Coronary anomalies that accompany many congenital cardiac lesions complicate repair, and the multiple suture lines required for repair may bleed after surgery in patients with coagulopathy.
Table 30–1 Differences Between Adult and Pediatric Cardiopulmonary Bypass
Parameter | Adult | Pediatric |
---|---|---|
Hypothermic temperature | Rarely <25-32° C | Commonly 15-20° C |
Circulatory arrest | Uncommon | Common |
Pump prime | ||
Dilutional effect on blood volume | 25%-33% | 200%-300% |
Additional additives | Blood, albumin | |
Perfusion pressure | 50-80 mm Hg | 20-50 mm Hg |
Influence of pH management | Minimal at moderate hypothermia | Marked at deep hypothermia |
Measured PaCO2 differences | 30-45 mm Hg | 20-80 mm Hg |
Glucose regulation | ||
Hypoglycemia | Rare; requires liver injury | Common ↓ glycogen stores |
Hyperglycemia | Frequent; insulin bolus/infusion | Less common; rebound ↓↓ glucose |
From Dinardo JA: Physiology and techniques of extracorporeal circulation in the pediatric patient. In Lake CL, Booker PD: Pediatric cardiac anesthesia, Philadelphia, 2005, Lippincott Williams & Wilkins, p 229.
Physiology of Cardiopulmonary Bypass
Hypothermia
Normothermia and moderate hypothermia are used in cases where the surgeon is able to safely cannulate both vena cavae in order to operate within the heart. This, of cours,e can be done even on the smallest of patients, bearing in mind that the cannulae may cause vena caval obstruction and impaired drainage that can adversely affect both cerebral and abdominal perfusion, independent of perfusion pressure. Deep hypothermia with reduced pump flow to as low as 25 to 50 mL/kg/min will allow the surgeon to perform corrective surgeries with only minimal return of blood to the surgical field while at the same time maintaining vital organ perfusion. DHCA is used for the repair of complex intracardiac lesions as well as reconstruction of the aorta. Once circulation has ceased, the surgeon is able to remove all cannulae and work in a completely bloodless and unobstructed field. An alternative for aortic surgeries is DHCA with low-flow antegrade cerebral perfusion with an arterial cannula in the innominate artery and drainage via a suction catheter or venous cannula in the right atrium/superior vena cava. Flow rates of between 20 and 50 mL/kg/min to a perfusion pressure of 30 to 50 mm Hg are used.8
The principal clinical effect of hypothermia is to reduce metabolic rate and molecular movement. As temperature is lowered, both basal and functional cellular metabolism are reduced, and the rate of adenosine triphosphate consumption is decreased. Whole body oxygen consumption decreases directly with body temperature. The cerebral metabolic rate (CMRO2) has been found to exponentially decrease as temperature decreases throughout the clinically relevant temperature range of 37° to 18° C.9,10 The reduction in CMRO2 has been calculated to be between 82% and 87% of baseline in both the canine model and the pediatric population.9 The relationship between temperature and metabolic rate is quantified experimentally by calculating a temperature coefficient known as the Q10. It is defined as the ratio of metabolic rates at two different temperatures separated by 10° C. In the adult population, this has been found to be 2.4 to 2.8, while in the pediatric population it is 3.65. This shows that in the pediatric population there is a greater decrease in metabolic rate as the core body temperature drops. Clinically, this translates to potentially greater brain and organ protection with hypothermic temperatures.
In contrast to the role that hypothermia plays in cerebral protection, it has less importance in protection of the myocardium; indeed, it has been suggested that electrical quiescence accounts for approximately 90% of myocardial protection at normothermia.11 It has been found that a decrease in perfusion temperature has a possible deleterious effect on the myocardium, as it leads to abnormal calcium and sodium handling. As Na+/K+ ATPase inhibition occurs with a falling temperature, it gives rise to an increase in intracellular sodium. This may then result in an increased activity of the Na+/Ca2+ exchanger, with an increase in intracellular calcium.12 This may play an important part in the development of an entity described as rapid cooling contracture, which is thought to play a role in postbypass myocardial dysfunction. This effect of cooling on myocardial performance may be felt more in neonates and infants, as their lower total body and cardiac masses make them susceptible to more rapid temperature changes.13
The adverse effects of rapid cooling have been demonstrated experimentally in the kidneys, liver, and lungs as well. In clinical practice, however, many variables are involved and there does not seem to be readily apparent injury to the patient from rapid cooling.14,15 It does appear that the neonate is able to tolerate the stress of profound hypothermia without difficulty. The reason for this may be the fact that neonates possess differential ionic channel density or have different membrane function when compared to older subjects. These theories remain speculative.
Pulsatile Versus Nonpulsatile Flow
The question whether pulsatile flow could improve outcome compared to nonpulsatile flow remains unanswered. The basis for this concern stems from the suggestion that nonpulsatile perfusion may be associated with microcirculatory dysfunction and thus may be important in the genesis of post-CPB myocardial, cerebral, and renal dysfunction.16 The desirability of pulsatile perfusion has been contemplated and studied since the introduction of extracorporeal circulation in the 1950s.
The difference between these two modes of perfusion is not simply the generation of a pulse pressure. Pulsatile flow also depends upon creation of an energy gradient and thus pump flow as well as arterial pressure must be included.17 This relationship was described by Shepard in 196618 and is known as the Energy Equivalent Pressure Formula:
Thus it is the ratio of the area under the hemodynamic power curve (∫fpdt) and the area under the pump flow curve (∫fdt), where f is the pump flow rate, p is the arterial pressure (in mm Hg), and dt indicates that the integration is performed over time (t). EEP is measured in mm Hg, and therefore a comparison can be made between it and mean arterial pressure (MAP). The difference between these two measurements is the energy generated by the bypass machine, be it a pulsatile or nonpulsatile device.17 This difference must then be applied to the surplus hemodynamic energy (SHE) formula:
The unit of measure of this formula is the erg, which measures energy. Pulsatile flow is best represented by an energy gradient rather than a pressure gradient. Pulsatile perfusion produces greater SHE units than nonpulsatile perfusion.19 This improved generation of energy units may translate into improved regional and global blood flow to vital organs.20 The converse is also true, that when there is no difference in energy level generated between pulsatile and nonpulsatile flow then there is no difference in vital organ blood flow (see Figure 30-2).21
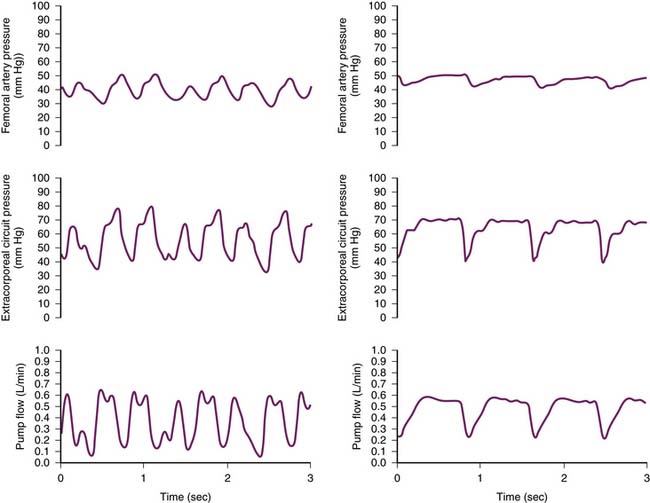
Figure 30–2 Pressure wave differences between pulsatile and nonpulsatile flow.
(Modified from Undar A, Masai T, et al: Effects of perfusion mode on regional and global organ blood flow in a neonatal piglet model, Ann Thorac Surg 68:1336-1343, 1999.)
Pulsatile flow appears to improve organ flow during CPB and function in the post-CPB period. In a pilot study of 50 patients, Alkan et al.22 described improved myocardial function in the pulsatile group based upon the observation that this group of patients required less inotropic support. The same investigators found equivalent findings in a follow-up study of 215 patients.20 This was similar to experimental findings of improved myocardial blood flow and function even after up to 60 minutes of DHCA.23
Cerebral blood flow patterns have also been studied and experimental animal models have been used to find differences in flow techniques. It is noted that both methods of perfusion show decreased flow when compared to pre-CPB. The findings showed that cerebral hemispheric, basal ganglia, brainstem, and cerebellar flows were better maintained in the pulsatile group. This was found at normothermic CPB, pre-DHCA, post-DHCA and even post-CPB. An important factor related to these findings is that cerebral vascular resistance is found to be lower under pulsatile perfusion during and after normothermic CPB.19 Interestingly, authors involved in the aforementioned work have performed other studies that do not agree with these findings but, despite this, they make a point of concluding that in their opinion pulsatile flow remains a better choice based upon its ability to generate more hemodynamic energy, which in turn may maintain the microcirculation to a greater degree.21,24
Postoperative ventilation and the effects of CPB on pulmonary function are important clinical markers in patients undergoing cardiac surgery. In their large clinical study, Alkan et al.20 found that time to extubation and length of ICU stay were significantly shorter in the pulsatile perfusion group, from which it can be concluded that pulmonary function was improved in this group. Renal function was also a marker analyzed in this study, and it was found that urine output was significantly improved in the pulsatile group, although the serum creatinine was similar in both groups. Indeed, it has been shown that pulsatile perfusion increased renal, and specifically renal cortical, flow. Pulsatile perfusion also played a role in decreasing edema and ischemic changes found in renal tubules, as compared to nonpulsatile flow.25
One of the biggest problems faced by clinicians when patients are placed on CPB is the inflammatory cascade that is initiated secondary to the exposure of patient’s blood to the foreign surface of the bypass circuit, surgical trauma, ischemia/reperfusion, hemodilution, hypothermia, and possibly changes related to the artificial nature of perfusion. The end result is organ dysfunction that affects the myocardial, pulmonary, hepatic, and renal systems. The release of cytokines from damaged endothelium is increased. The use of pulsatile cardiopulmonary bypass has been associated with a lower concentration of interleukin-8 (IL-8), leading authors to speculate that this may improve outcomes with regard to function and microcirculation of vital organs.22,26
Strategies for Blood Gas Management: Alpha-Stat and pH Stat
Another topic of considerable debate in pediatric CPB is the question of interpretation and management of pH, most specifically at deep hypothermia. In adult patients undergoing CPB, the commonest cause of brain injury is related to the delivery of embolic material to the cerebral vasculature. The etiology of brain injury in children is thought to result from hypoperfusion with cerebral hypoxia/ischemia during periods of low flow or DHCA.26 As has been mentioned in prior discussion, many pediatric cardiac operations are performed under conditions of decreased flow rates with moderate to deep hypothermia, with the aim of protecting against vital organ injury. It would thus follow that strategies that result in the lowest cerebral rate of oxygen consumption (CMRO2) would offer the best protection.
The temperature at which the pH is reported is of fundamental importance. Within the body, maintenance of electrochemical neutrality is necessary to ensure that cellular protein and enzymes function optimally. This neutrality occurs when there are equal concentrations of positively and negatively charged ions within aqueous solutions. As the temperature begins to drop, the dissociation constant of aqueous systems will increase, and thus the concentration of H+ begins to decrease. As pH is the inverse log of H+ and electrochemical neutrality must be maintained, the pH of the solution will increase. Electrochemical neutrality is also maintained within cells, and thus we can hypothesize that the pH within cells is a pH of neutrality. Hence we would then assume that the intracellular pH would change with a change in temperature to maintain neutrality. Analysis on ectothermic animals shows that intracellular pH remains at or near this pH of neutrality even as body temperature drops. For this to occur, there are two additional processes that occur. The first is a buffer system with a pK value close to that of the intracellular pH and whose pK changes as temperature changes. The buffering capacity of the imidazole of the amino acid histidine has these characteristics. Histidine is found commonly within intracellular proteins as well as enzymes. The term alpha refers to the degree of dissociation of the imidazole, i.e., the ratio of unprotonated to total imidazole; under normal conditions within the intracellular compartment, this has a value of 0.55. This dissociation (and hence the alpha value) will remain constant over a range of temperatures, as there is a proportional change of the neutral pH of water, the pKa of imidazole, and the tissue pH. The second necessary process is that the CO2 content of the blood must remain constant as body temperature drops. This is the alpha-stat condition. If, however, during hypothermia the pH remains constant, then the alpha number of the imidazole will change and thus there may be a change in protein structure or enzyme function. This is known as the pH-stat condition. In this management technique, in order for the pH to remain constant through the range of hypothermic temperatures, CO2 is added (Figure 30-3).
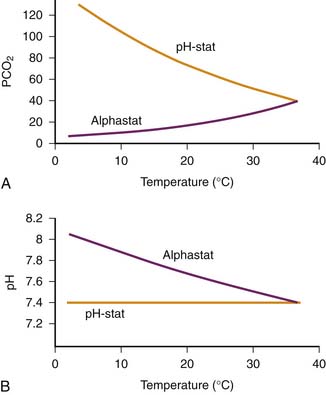
Figure 30–3 The effect of temperature on blood plasma pCO2 and pH.
(Modified from Tallman RD: Acid-base regulation, alpha-stat, and the emperor’s new clothes, J Cardiothorac Vasc Anesth 11:282-288, 1997.)
Into the 1990s, based upon adult studies and strategies, the alpha-stat technique was thought to have a better neuroprotective outcome. Despite the lack of prospective data, it gained widespread use amongst institutions performing cardiac surgery on children.26 In a neurodevelopmental follow up of 16 patients who had undergone Senning operations, Jonas and colleagues reviewed perfusion techniques and pH strategy. They found that the use of alpha-stat strategy prior to DHCA was associated with poorer developmental scores. The authors postulated that the lower cerebral blood flow associated with alpha-stat might result in inadequate cooling. This study, despite having the groups at different ages at the time of assessment and being underpowered with respect to numbers, hinted at a possible need to change strategies in the pediatric population.27 Another more devastating neurologic complication of pediatric cardiac surgery is the development of choreoathetosis. In two studies, including one from the above group, patients underwent DHCA and later went on to develop choreoathetosis. After analysis, apart from having the use of DHCA in common, it was found that all of the affected patients were managed using alpha-stat.28,29 Laboratory studies have demonstrated that the use of pH-stat appears to offer better cerebral protection. Neonatal piglets were used in these studies, with DHCA times varying from 60 to 90 minutes. In the studies referenced, the markers used to compare the two management strategies included microvascular diameter during cooling and rewarming, cerebral tissue oxygenation, brain cooling, and metabolic markers of ischemia, such as extrastriatal dopamine production.31,32 The effects on brain cooling include a faster rate of cooling which is uniform across all regions of the brain, especially the cortical white matter.33 The results of these showed pH-stat to be superior in all of the aforementioned categories in the cooling phases of DHCA as well as in the recovery phase. In the first prospective trial on the use of pH-stat management in the cooling phases of DHCA, du Plessis and colleagues found that there was a greater degree of cerebral cooling, as measured by tympanic membrane temperature, in the pH-stat group. This they attributed to increased cerebral blood flow, which appeared to be equally distributed throughout the brain. In keeping with these data, this group also had more rapid recovery of electroencephalographic (EEG) activity compared to those in whom alpha-stat management had been used. EEG-confirmed seizures were also increased twofold in the alpha-stat group.26 Although a section in this chapter will be dedicated to the discussion of monitoring with near-infrared spectroscopy (NIRS), studies suggest that the use of alpha-stat is associated with lower signals during cooling, indicating that this method results in insufficient cerebral blood flow and oxygenation.34,35 The next question to be answered will be whether this improved brain perfusion and cooling seen with pH-stat will translate into improved neurologic outcomes. Patients enrolled in this trial underwent neurodevelopmental testing at 1 year of age, using standardized tests. There was no difference in scores based on treatment group assignment. The authors concluded that the “use of alpha-stat versus pH-stat acid-base management strategy during reparative infant cardiac operations with deep hypothermic cardiopulmonary bypass was not consistently related to either improved or impaired early neurodevelopmental outcomes.”
The effects of pH/CO2 on other organ systems have also been studied. Myocardial protection is also obviously of great concern to the entire care team. In a study performed by Nagy et al. in which troponin I was used as the marker of myocardial injury, it was found across a spectrum of patients, including some with pulmonary hypertension and cyanotic congenital heart disease, that those in whom pH-stat was used had better myocardial performance after CPB.36 They postulated that pH-stat might induce a more even myocardial temperature during cooling due to hypercapnia-induced vasodilation. Oxygen delivery may also be improved as the oxygen-hemoglobin dissociation curve shifts rightward with the increase in CO3. Lastly, they suggested that the hypercapnia-induced inhibition of pyruvate dehydrogenase, which leads to a decrease in lactate production, may also be of great benefit. As a secondary outcome, their study also suggested that postoperative ventilation and length of ICU stay may be shorter in the pH-stat group. Improved myocardial performance had also been found in the du Plessis study, where there appeared to be a higher cardiac index within the first 18 hours after CPB with lower inotropic use, less hypotension, and less metabolic acidosis compared to the alpha-stat group. These patients also had significantly less mechanical ventilation and shorter ICU stays.26
Despite these advantages, there is concern that the use of pH-stat may result in a change in the internal pH (pHi) of cells that would lead to a prolonged depletion of high energy phosphates during rewarming, which in turn would lead to cellular damage. Experimental evidence, however, seems to suggest that the pHi of cells is regulated independently of the extracellular environment. Hiramatsu et al. showed that the pHi was alkalotic irrespective of the use of alpha-stat or pH-stat strategies during the cooling phase, but that it tended towards acidosis with the onset of DHCA.30 The data then suggested that there was a quicker recovery of both pHi and ATP using the pH-stat technique. As mentioned before, this was on the basis of a greater oxygen reserve secondary to increased blood flow and oxygen delivery in the cooling phase. In conclusion, it would seem that the evidence presented here would undoubtedly indicate that a prudent approach would be for the use of pH-stat management during the cooling phase of deep hypothermic CPB; indeed, there is also some evidence from these studies that this strategy should be employed in the rewarming phases as well. Many centers, including our own, use alpha-stat during rewarming with good clinical outcome
Neurologic Injury and Protection
The last 30 years have seen the advent of surgical techniques and management strategies that have changed a diagnosis from universally fatal to one that can be palliated well into adulthood. The challenge we now face is the long-term effects that these very techniques and strategies have on neurodevelopmental outcomes. In contrast to the adult population where neurologic injury commonly involves thromboembolic stroke, in the pediatric population the injury results in more subtle effects such as attention-deficit problems and difficulties with fine and gross motor control, visual motor integration, and executive functioning. The end result of these problems is school failure and low self-esteem. This “developmental signature” is similar to that seen in very low birth weight premature infants.37
It is quite alarming to note that long-term follow-up has found that neurodevelopmental impairment occurs in more than 50% of children who have cardiac surgery as newborns or young infants.38 Many studies of neurodevelopmental outcome after cardiac surgery in infancy have focused on injury during the intraoperative period as the likely cause of adverse outcomes. However, there is increasing evidence that brain development is abnormal in children with congenital heart disease (CHD), and injury can often be identified prior to surgery. Patient-specific factors such as genetic abnormalities, socioeconomic status, and maternal education are more important determinants of neurodevelopmental outcomes than are intraoperative management strategies.39 Box 30-2 highlights a number of factors that influence neurologic outcome.
Preoperative Factors
Neonates with CHD have a higher incidence of cerebral dysgenesis than those in the general population.40 The era of staged reconstruction of hypoplastic left heart syndrome began in the late 1970s. The diagnosis was fatal prior to that time. In a series of postmortem examinations from the early- to mid-1980s, 29% of the patients had either minor or major central nervous system (CNS) abnormalities. The absence of dysmorphic features did not preclude CNS abnormalities.41
Periventricular leukomalacia (PVL) is one of the most frequent and severe neuronal injuries specific to the neonatal brain. It is caused by necrosis of deep white matter adjacent to the lateral ventricles. The mechanism is thought to be related to hypoxic/ischemic injury to immature oligodendrocytes, the very cells responsible for myelination throughout the central nervous system. In early life, the brain undergoes an intensive period of neuronal development and axonal growth; thus the white matter of the brain is particularly susceptible to injury.40 It is during this period that the brain is susceptible to ischemia-reperfusion injury due to its fragile vascularity, high metabolic activity, and immature autoregulation. PVL occurs not only in the period surrounding CPB but also in premature infants and after such insults as hypoxia, hypoglycemia, and meningitis. The incidence in infants with CHD prior to cardiopulmonary bypass has been estimated to be between 16% and 25%,42,43 with this rate increasing to between 54% and 73%43,44 post-CPB, based upon magnetic resonance imaging (MRI) studies. Studies have been performed using both MRI and fetal ultrasound and have demonstrated altered cerebral blood flow in fetuses with congenital heart disease; this may therefore be a basis of abnormal brain development. MRI studies have also found alarmingly low cerebral blood flow in infants with CHD prior to surgery, in some cases less than 10 mL/100 g/min.45 Other associated factors include the presence of ductal-dependent blood flow with resultant diastolic hypotension that lowers cerebral perfusion, balloon atrial septostomy with possible embolic phenomena,46 and ongoing systemic inflammation due to endotoxemia from mesenteric hypoperfusion.38
An interesting genetic association has also been found. Apolipoprotein E (APOE) is an important lipoprotein that aids in cholesterol metabolism as well as being an important cofactor in neuronal repair. There are three common forms of APOE (E2, E3, and E4), which are encoded by three alleles (ε2, ε3, and ε4). Research has shown that the APOE genotype has a strong association as a determinant of neurologic recovery following CNS injury. The presence of the APOE ε2 allele is associated with lower Psychomotor Developmental Index (PDI) and Mental Developmental Index (MDI) scores when compared to age-matched normals. Other associated risk factors include a confirmed genetic syndrome, lower birth weight, small head circumference at 1 year, preoperative intubation, and history of ECMO or ventricular assist device.47,48
The intraoperative period is a time of great risk to the child. In two contrary sets of data, two apparently different neurologic outcomes were found. In a study performed in patients undergoing atrial septal defect closure either surgically or via catheter, there was a significant decrease in intelligence quotient in those undergoing surgery.49 However, preliminary data from another cohort failed to show a decrease in neurocognitive function in 41 patients with age ranges from 5 to 18 years exposed to CPB for closure of simple atrial or ventricular defects, or for simple valve surgery.
Factors that likely result in poorer neurodevelopmental outcomes include anesthetic-related issues encompassing a decrease in cardiac output with decreased oxygen delivery at the time of transition from negative to positive pressure ventilation, and also the negative inotropic effects of anesthetic agents. Hypoxia may worsen prior to establishment of CPB, particularly in those with ductal-dependent flow, as the surgeon dissects around the ductus causing vasospasm. Once on CPB, numerous factors may occur that may have an adverse effect. These include malposition of both the arterial and venous cannulae with resultant poor flow characteristics and venous drainage leading to cerebral hypoperfusion; embolic phenomena including air and thrombus (may occur despite meticulous circuit check, adequate anticoagulation, and de-airing of the heart once repair is undertaken); the inflammatory response induced by CPB; the effects of DHCA with ischemia-reperfusion injury and uneven cerebral cooling; hemodilution; pH management; and the effect of collateral steal of blood away from vital organs.38,40 Once weaning from CPB has taken place, it is the effect of continued myocardial dysfunction with decreased oxygen delivery and hypoxia that may lead to ongoing brain injury. The need for ECMO for postcardiotomy support (2% to 5% of patients)50 carries significant morbidity and mortality, with up to 50% of survivors showing neurodevelopmental problems.51
Neuromonitoring and Neuroprotection
In recent years, interest has been focused on neurologic monitoring with near-infrared spectroscopy (NIRS), transcranial Doppler, EEG, and jugular venous saturation alone or in combination, as opposed to using surrogate markers of perfusion pressure, mixed venous saturation, and acid-base status.52
NIRS provides a real-time, noninvasive assessment of regional cerebral oxygenation and has been validated with jugular venous saturation.52 In this method, a venous-weighted approximation of tissue oxygen saturation is measured with a venous:arterial blood ratio of 85:15. This technology uses modifications of Beer’s equation. Infrared light passes through the skin, penetrates through tissue, and then returns, whereupon allowing assessment of the oxyhemoglobin saturation of tissues at a depth of 2 cm. Subtraction of the shallow light path from the deep light path allows assessment of brain oxygenation.52 The INVOS 5100 (Somanetics, Inc, Troy, Mich.), which is approved by the FDA for use in adults and children, calculates a regional cerebral saturation index (rSO2i) by computing the ratio of oxyhemoglobin to total hemoglobin. It has a range of 15% to 95%. These monitors are best used as trend monitors, with a fall of 20% from baseline being significant.53 Factors that affect cerebral oxyhemoglobin saturation include cerebral perfusion pressure, cerebral blood flow, arterial oxyhemoglobin saturation, PaCO2, and cerebral metabolic rate. The baseline values have been quantified, with 70% being normal in adults. Studies of single-ventricle patients, and laboratory studies, have suggested that a saturation below 30% suggests dependence on anaerobic metabolism.54,55
NIRS has thus been used to guide a safe duration of DHCA, proper placement of CPB cannulae, and also to guide optimal flow during low-flow cerebral perfusion during deep hypothermia.56–58 Some authors have even suggested that the placement of a NIRS probe over the anterior abdominal wall or renal bed will give an idea of somatic flow that in conjunction with cerebral NIRS will give invaluable data with respect to adequacy of perfusion.59,60 EEG monitoring is theoretically attractive for the detection of hypoperfusion or ischemia on the basis of altered signals. It is, however, not routinely feasible, as the technology is bulky and the signals may be affected by electrical interference from electrocautery, patient temperature, anesthetic agents used, and CPB itself. EEG is, however, useful for quantification of anesthetic depth, occurrence of electrical silence with DHCA, and of course the presence of epileptiform activity in the postoperative period that may indicate neuronal damage.53
Transcranial Doppler (TCD) provides a real-time monitor of cerebral blood flow velocity and the occurrence of embolic phenomena during CPB. The instrument uses pulsed-wave ultrasound, usually at 2 MHz frequency. The units display the frequency of the Doppler signals; peak systolic and mean flow velocities (in centimeters per second); and the pulsatility index, which is the peak velocity minus the end-diastolic velocity divided by the mean velocity.61 The added advantage of the device is the identification of microemboli via high intensity transient signals displayed as an audible click for each event. The most consistent and reproducible technique is to monitor the middle cerebral artery through the temporal window, which is located superior to the zygoma and anterior to the tragus. Clinically, either an inflow arterial obstruction, which will be seen by a drop in the peak Doppler signal, or an outflow obstruction, which is diagnosed by a decrease in the diastolic flow signal, can be appreciated. The etiologies include malposition of the arterial cannula, arterial line obstruction, changes to perfusion pressure, and changes in the flow rates, or alternatively obstruction to venous drainage from the head by superior vena caval cannula malposition, kinking of the venous line, or after the creation of a superior cavopulmonary anastomosis.52
Jugular venous bulb saturation (SjO2), as its name implies, measures the saturation of the venous blood by the placement of a retrograde catheter into the jugular venous bulb located, approximately, just cephalad of the C1-C2 disc. The jugular veins drain almost all blood from the brain, but up to 6.6% of this blood comes from extracranial sources including the emissary and frontal veins and the facial vein, which contributes the most extracranial blood.62 Another potential anatomic problem with this monitoring technology is that blood does not drain equally into the two jugular venous bulbs. In the pediatric population, the use of SjO2 has been studied in the context of optimal cooling time in those undergoing DHCA. As the temperature drops, the brain will extract less oxygen as its metabolic demands fall. Under optimal conditions, as the temperature falls, the SjO2 rises, until the difference between the arterial saturation and the jugular venous saturation becomes minimal.63 At this point, it can be concluded that the metabolic rate of the brain has reached a nadir at which maximal protection is occurring. This technique is clearly invasive and thus studies have been performed comparing this technique to NIRS. These studies demonstrate that although there is a good correlation, certain limitations occur.64–67 These involve the accuracy of NIRS at low SjO2; NIRS may have difficulty reading values greater than 90% when cerebral metabolism is slowing, and has questionable accuracy at low flow rates. The best correlations between the modalities occur in patients who weigh less than 10 kg, because of their lesser scalp and skull thickness.65,66
Some centers use triple monitoring; that is, NIRS, EEG, and TCD. The advantage of this is that the monitoring is noninvasive and relatively easy to apply. Once an abnormality is detected, adjustments in patient positioning, cannula position, blood pressure, hemoglobin, PaCO2, and depth of anesthesia may be needed in the prevention of potential neurologic injury.38,52,53,61 In a study of triple monitoring on 250 patients, at first the investigators did not treat any changes that were observed, as this phase was an attempt to validate this triple monitoring. Twelve (26%) of 46 of these patients developed early postoperative neurologic problems inclusive of seizures, coma, hemiparesis, movement, vision or speech disorder. In the group in which an intervention was performed when a problem was found, the complication rate decreased to 7 out of 130 patients (6%). Of note in the study was that in the remaining 74 patients in whom no changes were detected, 5 patients had adverse outcomes. Of the monitoring modalities, EEG found 5% of the abnormalities, while NIRS and TCD found 58% and 37%, respectively.68 A recent meta-analysis of NIRS monitoring alone found it to be a useful adjunct, but that greater clinical relevance can be achieved by combining modalities. However, despite measuring regional cerebral saturation, there is a paucity of data suggesting improved neurologic outcome based upon these numbers alone.69
Box 30-3 illustrates a suggested strategy for neuroprotection and monitoring during CPB. A recent study failed to demonstrate an association between a low rSO2 during CPB and neurologic outcome.70 It did, however, show that there appeared to be an association between preoperative MRI findings of brain immaturity and outcome. This led the authors to suggest that the preoperative MRI finding of immaturity, which results in vulnerability to injury, may be a useful marker for long-term outcome.70
Neuroprotection must be continued well into the postoperative phase. We know that cerebral autoregulation is not normal in the immediate postoperative period and thus adequate perfusion is reliant on MAP. Important management strategies include avoiding alkalosis and hypocarbia as these may further decrease cerebral blood flow, and early treatment of hypoxia, hyperthermia, acid-base disturbance, and electrolyte abnormalities. It has been suggested that continued NIRS monitoring in the postoperative phase may be useful at guiding therapy to protect the brain, but its impact on neurodevelopmental outcomes remains unclear.38
Myocardial Protection
Experimental evidence suggests that neonatal and infantile myocardium will have an earlier accumulation of lactate that in turn inhibits glycolysis and the generation of ATP. The decreased ATP will prevent the uptake of calcium by the sarcoplasmic reticulum. As such, the immature myocardium that relies on glucose as its major energy substrate and extracellular calcium for excitation coupling is at a major disadvantage.71–73 The end result is earlier ischemic damage compared to the adult. Unfortunately, most patients operated on in infancy have associated cyanosis, myocardial hypertrophy, hypoxia, volume and pressure overload, and acidosis. Thus it is obvious that these factors, coupled with immature myocardial physiology, place these patients at an enormous disadvantage. The presence of large amounts of collateral flow associated with a number of congenital abnormalities will result in increased flow to the left heart that can result in the washing away of cardioplegia and constant myocardial warming. Either of these factors can result in inadequate myocardial protection.74 It has been found that up to 90% of patients who do not survive the perioperative period show some form of myocardial necrosis that exists in the absence of coronary artery obstruction. This necrosis may affect the entire subendocardium and lead to endocardial fibrosis with late myocardial dysfunction.75
The major factors that will aid in myocardial protection are electrical quiescence achieved by the infusion of cardioplegia into the aortic root after aortic crossclamping or directly into the coronary arteries, hypothermia, and the prevention of myocardial distension. Cardioplegia solution is infused, under the direction of the surgeon, by the perfusionist. The principles that guide the composition of the solution include (1) ability to produce rapid cardiac arrest, (2) hypothermia, (3) metabolic substrates, (4) appropriate pH, and (5) membrane stabilization (i.e., low Ca2+, Mg2+, steroids, O2 radical scavengers).76 Historically, crystalloid cardioplegia has been used. Apart from the high concentration of potassium that is responsible for the electrical quiescence, the concentrations of both calcium and magnesium have been found to be important. Ideally the solution should be hypocalcemic and hypermagnesemic. Experimental studies have shown that under hypoxic conditions (cyanotic heart disease), hypocalcemic cardioplegia allows for improved recovery from hypoxic damage. It has been found that high levels of intracellular Ca2+ during ischemia and reperfusion lead to increased cellular injury.76 The importance of high levels of magnesium is twofold: the prevention of calcium entry into hypoxic myocardial cells and the prevention of arrhythmia in the immediate post-bypass period.76 The two most widely used crystalloid cardioplegia solutions are the del Nido and St. Thomas solutions. The del Nido solution contains Na+ of 153 mmol/L, K+ of 24 mmol/L, Cl− of 132 mmol/L, Ca2+ of 0.4 mmol/L, Mg2+ of 6.2 mmol/L, lidocaine of 140 mg/L, and mannitol of 2.6 g/L. St. Thomas’ solution contains Na+ of 129 mmol/L, K+ of 16 mmol/L, Cl− of 140 mmol/L, Ca2+ of 1.2 mmol/L, Mg2+ of 16 mmol/L, and no mannitol or lidocaine. Each solution has its proponents and opponents.
Three phases of cardioplegia have been described: induction, maintenance, and reperfusion. Induction of cardioplegia covers the initial dose that results in electrical silence soon after initiation of CPB. All hearts receive noncoronary collateral blood flow via pericardial connections and, in some patients with aortopulmonary collaterals, this may be more significant. The presence, size, and number of these vessels may be variable, and thus the flow may be of sufficient volume to wash out the cardioplegia. This will result in an increase in myocardial temperature, as the collateral flow will bring blood to the heart that is at the temperature of the systemic perfusate and also, importantly, a possible return of electrical activity. This is the rationale to give intermittent doses of cardioplegia every 10 to 30 minutes.75–77 The periodic cardioplegia will result in 1) maintenance of arrest, 2) restoring myocardial hypothermia, 3) buffering acidosis and washing away acidic metabolites, 4) replenishing high-energy phosphates, and (5) counteracting edema with hyperosmolarity75,76 This is the maintenance phase of cardioplegia. The reperfusion phase is a critical time, as the mode of reperfusion appears to be more important than the duration of ischemia. In a study performed on 103 patients with both cyanotic and acyanotic congenital heart disease, one group received intermittent boluses of cold cardioplegia, while the second group received the same cardioplegia strategy, to which was added 300 mL/m2 of the same cardioplegia solution at 35° C, just prior to aortic crossclamp removal. This is known as terminal warm blood cardioplegia (TWBCP) and is often used in adult cardiac surgery. The use of TWBCP was associated with higher numbers of patients resuming spontaneous sinus rhythm, decreased inotropic use to wean from CPB (although nearly all patients in the study required inotropic support postoperatively in the ICU), improved lactate extraction, decreased troponin T, and decreased heart-type fatty acid binding protein. The latter two markers are indicative of cellular damage and necrosis. It would thus appear that the use of TWBCP may provide additional protection by improving myocardial aerobic energy metabolism and decreasing cellular damage.77
Three additional factors are of importance in optimal cardioplegia: adequate coronary distribution, the delivery pressure of cardioplegia solution, and whether one uses crystalloid versus blood cardioplegia. Although the pediatric patient generally does not suffer from coronary artery disease, the presence of aortic insufficiency, severe septal and ventricular hypertrophy, and the need to move the coronaries (arterial switch operation) are important considerations. Despite the requirement that the cardioplegia be delivered at a certain pressure to ensure adequate distribution, care must be taken not to perfuse the myocardium at too high a pressure as this may itself lead to myocardial and vascular endothelial cell damage, increased edema, and decreased ATP levels. A pressure of between 30 and 50 mm Hg has been shown to be adequate.75 The use of blood cardioplegia results in myocardial arrest in an oxygenated state, with no loss of ATP in the period of electrical activity prior to arrest. This is contrasted to the use of crystalloid where there is a high loss of ATP during this same period. Several authors and commentators have concluded that there appears to be a distinct advantage in the use of blood cardioplegia over crystalloid, be it at cold or warm temperatures.75–77,79–82 Some of these advantages included lower coronary sinus lactate, improved cardiac index, better echocardiographic evidence of left ventricular function, increased heat-shock protein (produced in myocardial cells in response to stress), and decreased troponin I release, which may correlate with less myocardial injury.
Pulmonary Effects of Cardiopulmonary Bypass
The institution of CPB may have deleterious effects on the lungs. It is, however, important to note that there is a group of patients who present to the operating room with preexisting abnormal pulmonary physiology. This is often caused by their preexisting cardiac condition. Some patients exhibit the ill effects of increased pulmonary blood flow due to unrestricted left-to-right shunt. The presentation at surgery is thus a spectrum of patients who have been successfully medically palliated with antifailure therapy, those who have failed medical therapy, and, worst case scenario, those with established pulmonary hypertension. This latter possibility is commonly found in those with trisomy 21 who are operated upon at or after 6 months of age. The pulmonary hypertension occurs due to unrestricted pulmonary blood flow that results in hypertrophic changes to the media of the pulmonary arteries. This places these patients at a clear disadvantage at the weaning phase of CPB. In some, this may be severe enough that the patient may suffer acute right ventricular failure, resulting in a failure to wean from CPB and thus necessitating the use of acute extracorporeal support. Other patients in whom vascular reactivity and pulmonary hypertension may be an issue during weaning and separation from cardiopulmonary bypass include neonates, by virtue of their pulmonary vasoreactivity, a carryover from the fetal circulation, and those patients in whom there is an obstruction to pulmonary venous drainage, be it at the level of the pulmonary veins or due to restrictive atrial septae associated with left-sided obstructive lesions. The lungs may be a source as well as a target of the inflammatory response associated with CPB.83 The result of this is capillary leak and extravasation of fluid into the alveolar spaces with resultant poor oxygen exchange and a detrimental effect on pulmonary mechanics. Etiologic factors associated with capillary leak include hemodilution with resultant decrease in oncotic pressure, ischemia-reperfusion injury, and the sequestration of activated neutrophils, cytokines, complement, and leukotrienes within the pulmonary vascular bed during the period of CPB. At the onset of CPB, perfusion will cease through the pulmonary bed, and that in turn leads to a decrease in alveolar stability.84 Clinical studies have also shown that there are changes to functional residual capacity (FRC) during CPB. Initially there is an increase in FRC as the chest cavity is opened. This will, however, change to a decrease with the cessation of pulmonary flow and alveolar collapse. There is a further decease in FRC with chest closure, continued inflammatory effects, and the presence of extravasated fluid.84 It can thus be appreciated that all these factors lead to the inevitable decrease in PaO2 encountered in the immediate postbypass period.
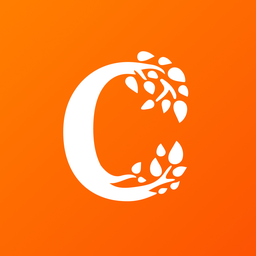
Full access? Get Clinical Tree
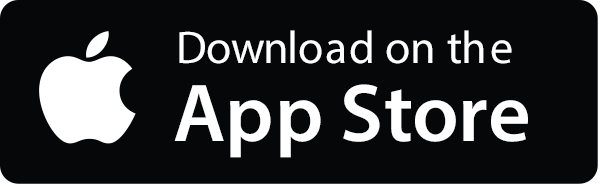
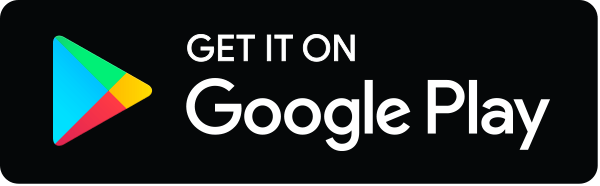