Brain metabolism involves both the production and the utilization of energy; catabolism is the breakdown and anabolism is the synthesis of components and molecules in the cells. For energy formation the main catabolic process is the breakdown of glucose with the ultimate formation of high-energy phosphate in the form of adenosine triphosphate (ATP). Other catabolic processes break down structural and enzymatic proteins, lipids, and carbohydrates; these processes are necessary to replace damaged and nonfunctional molecules. These molecules are resynthesized by anabolic processes that renew the cells and maintain optimal function. Cellular function also requires the maintenance of ionic homeostasis, which for neurons requires a large amount of energy. The pathophysiologic mechanisms of brain injury are incompletely understood but ultimately represent a failure of anabolic processes to maintain normal cell function. In this chapter we explore the putative mechanisms of brain injury. The causes of neuronal damage are multifaceted, and one pathway alone cannot explain how the injury occurs. Some pathophysiologic mechanisms are common to damage caused by ischemic, epileptogenic, and traumatic injury, whereas others are discrete for each of these processes. This review focuses on some common triggers of neuronal damage, such as altered ionic gradients, and explores how they in turn lead to long-term damage. We also discuss pharmacologic agents and clinical procedures that may lead to a reduction in long-term brain damage.
Brain metabolism
The main substance used for energy production in the brain is glucose. Because glucose is not freely permeable across the blood–brain barrier, it requires a transporter to enter the brain. This transporter does not require energy and can move glucose only down its concentration gradient, from a higher to a lower concentration. Normally the blood levels of glucose are well regulated so glucose concentrations in the brain are adequate; however, if blood levels of glucose fall the supply of glucose cannot meet the energy requirements of the brain. Thus adequate blood glucose levels are critical for normal brain activity. During insulin shock or other conditions that cause a reduction in blood glucose, unconsciousness can result from insufficient energy due to low brain glucose levels. When glucose and oxygen levels are sufficient, glucose is metabolized to pyruvate in the glycolytic pathway ( Fig. 1.1 ). This biochemical process generates ATP from adenosine diphosphate (ADP) and inorganic phosphate and produces nicotinamide adenine dinucleotide reduced (NADH) from nicotinamide adenine dinucleotide (NAD + ). Pyruvate from this reaction then enters the citric acid cycle which, with regard to energy production, primarily generates NADH from NAD + . The mitochondria use oxygen to couple the conversion of NADH back to NAD + with the production of ATP from ADP and inorganic phosphate. This process, called oxidative phosphorylation , forms three ATP molecules for each NADH converted and yields a maximum of 38 ATP molecules for each glucose molecule metabolized. Because numerous parts of this pathway supply other metabolic requirements, such as amino acid synthesis and the formation of reducing equivalents for other synthetic pathways, the normal yield of this energy pathway is approximately 30 to 35 ATP molecules for each glucose molecule.

This pathway requires oxygen; if oxygen is not present the mitochondria can neither make ATP nor regenerate NAD + from NADH. The metabolism of glucose requires NAD + as a cofactor and is blocked in its absence. Thus, in the absence of oxygen, glycolysis proceeds by a modified pathway termed “anaerobic glycolysis”; this modification involves the conversion of pyruvate to lactate, regenerating NAD + . This process produces hydrogen ion, which may accentuate neuronal damage if the intracellular pH falls. A major problem with anaerobic glycolysis, in addition to lowering pH, is that only two molecules of ATP are formed for each molecule of glucose metabolized. This level of ATP production is insufficient to meet the brain’s energy needs. In addition, ischemia curtails the supply of glucose so even anaerobic glycolysis is blocked.
When the oxygen supply to a neuron is reduced, mechanisms that reduce and/or slow the fall in ATP levels include the following: (1) the utilization of phosphocreatine stores (a high-energy phosphate that can donate its energy to maintain ATP levels), (2) the production of ATP at low levels by anaerobic glycolysis, and (3) a rapid cessation of spontaneous electrophysiologic activity.
Cellular processes that require energy
Pumping ions across the cell membrane is the largest energy requirement in the brain. The sodium, potassium, and calcium concentrations in a neuron are maintained against large electrochemical gradients with respect to the outside of the cell. When sodium (Na), calcium (Ca) and potassium (K) are mentioned throughout the chapter we are referring to their ionic form (Na + , Ca ++ and K + ); this is the only form of these compounds that is present in living cells. When a neuron is not excited, there are slow leaks of potassium out of the cells and of sodium into the cells. The resting potential of a neuron depends mainly on the electrochemical equilibrium potential for potassium, which in most neurons is approximately −94 mV. There is some permeability to sodium and calcium so the resting potential for a neuron is usually −60 to −70 mV. Because the cell’s membrane potential is not equal to the equilibrium potential for an ion, there is leakage of ions down their electrochemical gradients. If this leakage were not corrected by energy-dependent ion pumps, the membrane potential would fall to 0 mV and the cell would depolarize and die. The ion pumps fall into two major categories: (1) those that use ATP directly to pump ions and (2) those that use the energy of the Na gradient to cotransport another ion or molecule. The ultimate energy for the latter pumps comes from ATP via the Na/K ATPase, which transports Na ions and maintains the energy gradient of Na; examples of these exchange pumps include the Na/Ca, the Na/H and the Na/glutamate transporters. Examples of the former category of pump are the Na/K ATPase, the major user of energy in neurons, and the Ca ATPase. The primary ion pumps that directly use ATP are important because they establish the electrochemical gradients necessary for the secondary pumps, the ion exchange pumps, to work in the desired direction. Indeed, during ischemia these pumps do not have enough energy to operate, and this condition is a primary cause of neuronal depolarization and cell death. Neuronal activity markedly increases the flow of sodium, potassium, and calcium by opening Na, K, and Ca ion channels; this opening raises the rate of ion pumping required to maintain normal cellular ion concentrations. Because ion pumping uses ATP as an energy source, the ATP requirement of active neurons is greater than that of unexcited neurons. Approximately 60% of the energy the brain uses is required for functional activity, and the remainder is used to maintain cellular integrity. Anesthetics reduce neuronal activity and thereby ATP utilization by functional activity, but they do not reduce the energy required for the integrity of the brain. If energy production does not meet the demand of energy use in the brain, the neurons become first unexcitable and then irreversibly damaged.
Neurons require energy to maintain their structure and internal function. Each cell’s membranes, internal organelles, and cytoplasm are made of carbohydrates, lipids, and proteins that require energy for their synthesis. Ion channels, enzymes, and cell structural components are important protein molecules that are continuously formed, modified, and broken down in the cell. If ATP is not available, protein synthesis cannot continue, and the neuron will die. Carbohydrates and lipids are also continuously synthesized and degraded in normally functioning neurons; their metabolism also requires energy. Most cellular synthesis takes place in the cell body, and energy is required for transport of components down the axon to the nerve terminals. Thus, energy is required to maintain the integrity of neurons even in the absence of electrophysiologic activity.
Cellular processes that require energy
Pumping ions across the cell membrane is the largest energy requirement in the brain. The sodium, potassium, and calcium concentrations in a neuron are maintained against large electrochemical gradients with respect to the outside of the cell. When sodium (Na), calcium (Ca) and potassium (K) are mentioned throughout the chapter we are referring to their ionic form (Na + , Ca ++ and K + ); this is the only form of these compounds that is present in living cells. When a neuron is not excited, there are slow leaks of potassium out of the cells and of sodium into the cells. The resting potential of a neuron depends mainly on the electrochemical equilibrium potential for potassium, which in most neurons is approximately −94 mV. There is some permeability to sodium and calcium so the resting potential for a neuron is usually −60 to −70 mV. Because the cell’s membrane potential is not equal to the equilibrium potential for an ion, there is leakage of ions down their electrochemical gradients. If this leakage were not corrected by energy-dependent ion pumps, the membrane potential would fall to 0 mV and the cell would depolarize and die. The ion pumps fall into two major categories: (1) those that use ATP directly to pump ions and (2) those that use the energy of the Na gradient to cotransport another ion or molecule. The ultimate energy for the latter pumps comes from ATP via the Na/K ATPase, which transports Na ions and maintains the energy gradient of Na; examples of these exchange pumps include the Na/Ca, the Na/H and the Na/glutamate transporters. Examples of the former category of pump are the Na/K ATPase, the major user of energy in neurons, and the Ca ATPase. The primary ion pumps that directly use ATP are important because they establish the electrochemical gradients necessary for the secondary pumps, the ion exchange pumps, to work in the desired direction. Indeed, during ischemia these pumps do not have enough energy to operate, and this condition is a primary cause of neuronal depolarization and cell death. Neuronal activity markedly increases the flow of sodium, potassium, and calcium by opening Na, K, and Ca ion channels; this opening raises the rate of ion pumping required to maintain normal cellular ion concentrations. Because ion pumping uses ATP as an energy source, the ATP requirement of active neurons is greater than that of unexcited neurons. Approximately 60% of the energy the brain uses is required for functional activity, and the remainder is used to maintain cellular integrity. Anesthetics reduce neuronal activity and thereby ATP utilization by functional activity, but they do not reduce the energy required for the integrity of the brain. If energy production does not meet the demand of energy use in the brain, the neurons become first unexcitable and then irreversibly damaged.
Neurons require energy to maintain their structure and internal function. Each cell’s membranes, internal organelles, and cytoplasm are made of carbohydrates, lipids, and proteins that require energy for their synthesis. Ion channels, enzymes, and cell structural components are important protein molecules that are continuously formed, modified, and broken down in the cell. If ATP is not available, protein synthesis cannot continue, and the neuron will die. Carbohydrates and lipids are also continuously synthesized and degraded in normally functioning neurons; their metabolism also requires energy. Most cellular synthesis takes place in the cell body, and energy is required for transport of components down the axon to the nerve terminals. Thus, energy is required to maintain the integrity of neurons even in the absence of electrophysiologic activity.
Neuroanatomy
The brain is regionally differentiated structurally and functionally; this section will provide an overview of the functionality of the different brain regions. This is important with regard to stroke, since when an artery is blocked the function of the neurons in the region perfused by that artery is compromised. The details of the neuroanatomy and neurophysiology of the brain would require a book of its own; two that are recommended for detail are Clinical Neuroanatomy , by RS Snell and Neurophysiology and Principles of Neural Science by Kandel et al. ,
The cerebral cortex has four main lobes on each side: the frontal, parietal, occipital, and temporal lobes ( Fig. 1.2 ). Sensory pathways from one side of the body cross the midline and provide input to the opposite somatosensory cortex. Motor pathways that originate from the motor cortex on one side decussate in the medulla, travel down the spinal cord in the lateral corticospinal tracts, synapse on ventral motor neurons in the gray matter of the cord and deliver motor output to the opposite side of the body. The anterior part of the frontal lobe (prefrontal area) influences personality, orientation, concentration, and judgment; it is important for directing intellectual activity towards a goal. The precentral gyrus of the frontal lobe is the primary motor cortex, has output to the motor neurons in the spinal cord, and controls fine movement. Premotor association areas are located rostral to it and receive input from other motor areas of the brain, such as the basal ganglia, cerebellum, and red nucleus. Thus the premotor and motor cortex are responsible for integrating input from motor areas throughout the brain leading to purposeful movement. Adjacent to the precentral gyrus, across the central sulcus is the postcentral gyrus of the parietal lobe; this is the primary somatosensory cortex and receives information about fine touch. Posterior to the postcentral gyrus are the somatosensory association areas which help interpret and analyze touch sensations. All primary sensory areas of the brain have sensory association areas which further analyze and interpret these signals. The temporal lobe is located below the frontal and parietal lobe and contains the primary auditory and auditory association areas. One hemisphere in the brain is considered dominant and one particular area in it is important for the interpretation of language and the production of speech; this area has been labelled Wernicke’s area. Wernicke’s area is of critical importance and lesions in it lead to profound aphasia; it is generally considered to include the posterior part of superior temporal gyrus and the angular gyrus in the dominant cerebral hemisphere. The angular gyrus is an important multimodal association area in the parietal lobe adjacent to the temporal lobe. Multimodal association areas analyze input from single sensory association areas and provide complex analysis of the inputs and determine the response to complex stimuli. Wernicke’s area of the brain is most carefully mapped out during neurosurgery and damage to it is assiduously avoided if possible. It is supplied by the middle cerebral artery and there are profound deficits following occlusion of this artery due to ischemic stroke. Lesions in this area are isolating to the person and he/she cannot communicate or understand verbal or written communication. This area directly activates Broca’s area in the frontal lobe, a premotor speech area. Lesions to the parietal lobe of the nondominant hemisphere lead to visuospatial deficits and hemi-neglect (ignoring half of external space).
The thalamus is located medially and is an important relay center for information to and from the cerebral cortex. The hypothalamus, located below the thalamus, is important for a number of regulatory functions of the body such as hunger, thirst, and temperature regulation and the hypothalamus integrates behavioral and motivational activity from the limbic system with autonomic responses. The limbic system includes limbic cortex, hippocampus, and amygdala and is associated with feelings of reward and punishment, emotional behavior, learning, and memory. The hippocampus and the medial temporal lobe are important for long-term memory formation; the amygdala conveys the emotional content of memory. The basal ganglia are also located medial to the cortex and are important for motor function and the initiation of movements. Parkinson’s disease is due to lesions in the substantia nigra, a dopaminergic area, leading to resting tremor and bradykinesia. Dementia is a nonmotor correlate of basal ganglia diseases and indicates these areas, primarily thought to be motor, can also profoundly influence behavior.
The cerebellum is located above the brainstem and plays an important role in rapid learned motor activity as well as postural control; it receives input from the motor cortex and proprioceptive feedback from the body to compare the intended movement with the actual movement caused by the muscles. It is important to know that the lateral cerebellum is not crossed and controls the same side of the body; e.g., the right cerebellum controls muscles on the right side of the body, which is also controlled by the left motor cortex. Thus information from the cerebellar cortex crosses the midline on its way to the cerebral cortex.
The brainstem consists of the midbrain, pons, and medulla and is structurally continuous with the spinal cord. The cranial nerves III to XII originate from the brainstem and/or brainstem nuclei. Ascending and descending neuronal pathways traverse this part of the brain and synapse with neurons in it; this area contains the reticular formation and the reticular activating system, an area responsible for maintaining alertness and consciousness. This area is important for the control of blood pressure, heart rate, breathing, swallowing, and other bodily functions. Lesions in this area can lead to coma or rapid death.
The spinal cord allows the brain and the body to communicate and contains ascending sensory pathways and descending motor pathways. The anterolateral spinothalamic tracts convey crude touch, temperature, and pain; they enter the gray matter of the cord, synapse in the dorsal horn; the axons of the postsynaptic neurons cross the midline and ascend the spinal cord to the brainstem and thalamus in the anterolateral tracts. The dorsal columns convey fine touch and proprioception and ascend the cord on the same side of the body and cross the midline after first synapsing in nuclei in the medulla. The final destination of these axons is the thalamus and information is relayed from there to the somatosensory cortex on the postcentral gyrus. The dorsal column axons also send branches into the spinal cord at or near to the level of the spinal cord they enter. The spinal cord has neuronal circuitry that modifies input to the brain and also mediates local reflexes such as withdrawal from pain and the control of muscle tension and tone.
Pathophysiology
Ischemia
When the blood supply to the brain is limited, ischemic damage to neurons can occur; the brain is the organ most sensitive to ischemic damage. The area of the brain corresponding to the territory of the cerebral artery blocked determines what functions are altered or lost subsequent to focal ischemia; this corresponds to the functional anatomy described in the previous section. The neurons in the ischemic areas are damaged by the loss of energy; the rest of this section describes the cellular events subsequent to ischemia that lead to this damage.
The central event precipitating damage by hypoxia or ischemia is reduced energy production due to blockage of oxidative phosphorylation. This causes ATP production per molecule of glucose to be reduced by 95%. At this rate of production, ATP levels fall, leading to the loss of energy-dependent homeostatic mechanisms. Additionally, during ischemia the supply of glucose is interrupted, as is the washout of metabolites. The activity of ATP-dependent ion pumps is reduced and the intracellular levels of sodium and calcium increase, whereas intracellular potassium levels decrease ( Fig. 1.3 ). These ion changes cause the neurons to depolarize and release excitatory amino acids such as glutamate. In addition, glutamate is released from neurons owing to the reversal of the glutamate transporter, which pumps glutamate into the extracellular compartment when the cellular sodium and potassium ion gradients are disrupted. High levels of glutamate further depolarize the neurons by activating AMPA (α-amino-3-hydroxyl-5-methyl-4-isoxazole-propionate) and NMDA ( N -methyl- d -aspartate) receptors, increasing sodium and potassium ion conductance. The NMDA receptor also allows calcium to enter, triggering additional damaging pathways. Glutamate activates metabotropic receptors, which via second-messenger systems can increase the release of calcium from intracellular stores and activate other biochemical processes. The damage due to excess glutamate has been termed excitotoxicity and is caused by activation of glutamate receptors and the accompanying ionic and biochemical changes.
In addition to increased influx through membrane channels, cytosolic calcium is increased through reduced calcium pumping from the cell and the enhanced release of calcium from intracellular organelles such as the endoplasmic reticulum ( Fig. 1.4 ). The high cytoplasmic calcium level is thought to trigger a number of events that lead to the ischemic damage. These include increasing the activity of proteases and phospholipases. Phospholipases raise the levels of free fatty acids, such as arachidonic acid, and free radicals. Free radicals are also generated by incomplete mitochondrial oxidation. One of the most damaging free radicals is peroxynitrite, which is formed by the combination of nitric oxide and another free radical. Free radicals are known to damage proteins and lipids, whereas free fatty acids interfere with membrane function. There is a buildup of lactate and hydrogen ions during ischemia, which lowers the intracellular pH and this can lead to further formation of free radicals. All of these processes, coupled with the reduced ability to synthesize proteins and lipids, contribute to the irreversible damage that occurs with ischemia ( Box 1.1 ).
Triggers
Adenosine triphosphate ↓
Extracellular potassium ↑
Intracellular sodium ↑
Intracellular calcium ↑
Free radical levels ↑
Depolarization ↑
Glutamate level ↑
Effectors
Protease activity ↑
Free radical action ↑
DNA damage ↑
Phospholipase activity ↑
Mitochondrial factors ↑ (cytochrome c → caspase activation)
Critical Functional Changes
Mitochondrial damage ↑
Apoptotic cascade activation ↑
Antiapoptotic factors ↓
Protein damage ↑
Protein synthesis ↓
Cytoskeletal damage ↑
End Stage
Apoptosis ↑ (programmed cell death)
Necrosis ↑ (cell disintegration)
↑, increases; ↓, decreases; →, leads to
Additionally, phospholipase activation leads to the production of excess arachidonic acid, which upon reoxygenation can form eicosanoids, including thromboxane, prostaglandins, and leukotrienes. These substances can cause strong vasoconstriction, reduce blood flow in the postischemic period, alter the blood–brain barrier, and enhance free radical formation after reperfusion. ,
Procedures that protect against ischemic damage should interfere with the cellular changes brought on by ischemia ( Box 1.2 ). In addition to these direct triggering events, there is long-term damage that becomes apparent hours and days after the ischemic insult. Some of this delayed damage is necrotic and the lysis of the cells causes microglial activation. Lymphocytes, polymorphonuclear cells, and macrophages can invade the central nervous system, leading to additional damage. , Although histamine receptor activation is generally associated with immune system activation, the histamine receptor involved with this is the H 1 receptor. In the central nervous system, the H 2 receptor is the one primarily activated, and it reduces immunologic processes and improves recovery from ischemia. , Blocking immune system activation can reduce damage. It is clear there is also programmed cell death as a result of the insult. This apoptotic programmed cell death, which is similar to the cell death that occurs during neuronal development, can continue days after the initial insult.
Vascular Changes
Vasospasm
Red cell sludging
Hypoperfusion
Platelet aggregation
Endothelial injury
Leukocyte-endothelial adhesion
Blood–brain barrier disruption
Neuronal Changes
Adenosine triphosphate reduction
Sodium influx
Potassium efflux
Intracellular acidosis
High cellular calcium concentrations
Calcium-activated proteases
Caspase activation
Phospholipase activation
Arachidonic acid formation and breakdown
Free radical production
Excitatory amino acid release
Disruption of ion and amino acid transporters
Autophagy
Apoptosis
Necrosis
Necrosis versus Apoptosis
There are two major processes leading to neuronal death. The first, necrosis, is due to a more severe insult in which mitochondrial function is lost; it is characterized by a disintegration of the cell and an activation of microglia and the immune response. The immune response and inflammation activate and recruit neutrophils and macrophages, which produce free radicals and damage adjacent neurons. This process expands the lesion in volume and time, allowing for continued and expanded neuronal damage. In the second, apoptosis, the cell dies without breaking apart and there is no microglial or immune system involvement with the potential for excess damage to adjacent neurons. This process is frequently delayed and can lead to the activation of immediate early genes (IEGs) such as c-Jun and c-Fos; these genes are thought to affect gene expression and lead to the production of apoptotic or antiapoptotic proteins, which determine whether the neurons will survive or die. , One set of proteins that lead to neuronal death are the cysteine proteinases, referred to as caspases . These enzymes are expressed as proenzymes, which undergo proteolytic processing to yield active enzymes that degrade important proteins in the cell ( Fig. 1.5 ). , There are both intrinsic and extrinsic pathways to activate caspases and apoptosis, Fig. 1.5 shows the intrinsic pathway activated by mitochondrial cytochrome c release. In addition cell death receptors on the neuron membrane may be activated by death factors such as Fas ligand or tumor necrosis factor, which directly activate caspases. The final apoptotic pathway to cell death converges and is the same for both the intrinsic or extrinsically activated pathways. Blockade of caspases has been shown to block apoptosis. Because these enzymes are now known to be present as proenzymes before ischemia, new protein synthesis is not needed to induce apoptosis. However, proapoptotic proteins are synthesized under certain conditions, and their synthesis may lead to delayed neuronal cell death. Another set of proteins can be induced that block apoptosis and promote neuronal survival after ischemia; examples of these proteins are neuronal apoptosis inhibitory protein, heat shock proteins, and certain antiapoptotic Bcl-2 family proteins. , Thus the fate of ischemic neurons rests on the balance between apoptotic inhibitory and activating processes ( Fig. 1.6 ). , The synthesis of certain trophic factors can improve neuronal survival by inhibiting apoptosis (see Fig. 1.5 ). The activation and release of certain cytokines, such as tumor necrosis factor and interleukin-1β, are thought to be damaging. ,
Thus necrosis and apoptosis can be contrasted, with the former being a result of more severe ischemia and leading to the damage of adjacent tissue ( Fig. 1.7 ). Apoptosis is subject to modulation, so once started down the apoptotic pathway, cells have a chance of being rescued by trophic substances (see Fig. 1.6 ).
Global versus Focal Ischemia
Ischemia can be either global or focal in nature; an example of the former would be cardiac arrest, and of the latter, localized stroke. Although the mechanisms leading to neuronal damage are probably similar for the two types of ischemia, there are important distinctions between them. In focal ischemia there are three regions. The first region, called the ischemic core, receives no blood flow and responds in the same way as globally ischemic tissue; the second region, called the penumbra , receives collateral flow and is partially ischemic; the third region is normally perfused. If the insult is maintained for a prolonged period, the neurons in the penumbra die and the infarct (ischemic core) increases in size. More neurons in the penumbra region survive if collateral blood flow is increased or if reperfusion is established in a timely manner by opening the blocked vessel. With total global ischemia, the time until the circulation is re-established is critical, and only very short ischemic times (on the order of minutes) are survivable. The selective neurologic damage after survival subsequent to global ischemia is mainly due to the differential sensitivity of certain neurons and brain regions. The hippocampus, especially the cornu ammonis 1 (CA1) pyramidal cell region, is extremely vulnerable to ischemic damage; loss of learning and memory is common after global ischemia and hypoxia. , Other areas of enhanced sensitivity to global ischemia and hypoxia are the caudate, and putamen, as well as certain areas of the cerebellum and cerebral cortex. ,
Genetic Influences on Neuronal Damage
Genetic factors play an important role in an individual’s susceptibility to ischemic stroke. Both environmental (such as diet and stress) and genetic factors combine to determine the risk of stroke. A study of the Icelandic population found that polymorphisms (genetic changes) in genetic locus ALOX5AP, which encodes 5-lipoxygenase–activating protein, and PDE4D, which encodes phosphodiesterase 4D, increase the susceptibility to stroke. , In addition, polymorphisms of both apolipoprotein B and apolipoprotein E have been found to enhance the susceptibility to stroke. , The genetic factors could target neuronal risk but more likely raise the vascular risk, which is associated with an increase in both stroke and cardiac disease. If a patient’s genetic susceptibility to injury were known, it would be possible to choose therapeutic strategies individually for the patient to improve outcome.
Genetic factors have been shown to influence cardiovascular risk, particularly with respect to hyperlipidemia; controlling these factors with statins not only reduces cardiac disease but also reduces cerebral vascular disease and stroke rates.
Potential treatments for cerebral ischemia
Reperfusion Strategies
The goal for treatment of ischemic stroke is to achieve prompt restoration of perfusion and to preserve brain tissue in the ischemic penumbra. Reperfusion therapies include intravenous thrombolysis, intra-arterial thrombolysis, and endovascular mechanical thrombectomy. Comprehensive guidelines for early management of acute ischemic stroke were updated in 2013; this chapter can only very briefly summarize some of these important guidelines. Throughout this section recommendations that are not directly referenced are from this paper, any deviation from these guidelines will be explicitly stated. The most successful technique for improving recovery from embolic stroke is prompt restoration of spontaneous perfusion as soon as possible after the onset of a stroke. To date, despite recent evidence that for large vessel occlusion, intracranial mechanical thrombectomy is superior to pharmacological intervention alone, the only U.S. Food and Drug Administration (FDA)-approved method is the use of the thrombolytic agent, recombinant tissue plasminogen activator (rtPA); as one would predict, the effects of rtPA would markedly worsen hemorrhagic stroke. , , Thus detecting, classifying, and treating stroke rapidly after its onset is critical to a successful outcome. Thrombolytics cannot be used in patients with a high risk of bleeding; such as those with head trauma, recent surgery or with reduced clotting ability due to drugs such as warfarin, direct thrombin inhibitors and direct factor Xa inhibitors. Warfarin treated patients with an INR below 1.4 can be given rtPA treatment; however, the newer agents create a problem since the ability to assess coagulation function in patients taking these drugs is difficult and recommendations are under review.
The major side effect of rtPA is intracerebral hemorrhage, which can be devastating. It is essential that a noncontrast computed tomography scan be performed and analyzed shortly after patient presentation to the hospital in order to rule out a hemorrhagic stroke, because rtPA must be administered within 3 hours of an occlusive stroke onset to be effective. The guidelines from the AHA/ASA indicate that rtPA can be given to patients between 3 and 4.5 hours of stroke onset if patients are less than 80 years of age, not taking oral anticoagulants, and without a history of stroke and diabetes; this recommendation is not an FDA indication for rtPA. It is clear that the sooner rtPA is given the better the outcome is likely to be.
It is of key importance when exploring additional therapies to identify patients in whom an area of reduced perfusion has not progressed to irreversible neuronal damage. These studies use advanced imaging to identify at-risk tissue (penumbra) that can still be salvaged if reperfusion can be established. Diffusion-weighted magnetic resonance imaging (DWI) identifies core ischemic areas where water has shifted into the intracellular compartment and has reduced diffusibility. Areas that have not yet converted to core ischemia can potentially be rescued from irreversible damage. Perfusion-weighted magnetic resonance imaging indicates regions with reduced perfusion that will ultimately proceed to irreversible damage if not reperfused. The ratio of penumbral ischemic tissue volume to infarcted core volume is named penumbral mismatch. However, due to the importance of rapid reperfusion and the delay in carrying out MRI imaging, current practice uses multiphase CT angiography to determine if a large vessel is occluded, the location of the occlusion, and an assessment of either core-infarct volume or penumbral mismatch. Since rtPA is less effective and intra-arterial mechanical clot removal techniques are more effective for large occlusions, mechanical thrombectomy should be considered rapidly; with all reperfusion techniques an increase of the time to reperfusion equals increased brain tissue loss. A region with reduced perfusion that has not yet progressed to irreversible damage will benefit from endovascular reperfusion therapy.
The Merci Retriever (Concentric Medical, Inc., Mountain View, CA), the Penumbra System (Penumbra, Inc., Alameda, CA), the Solitaire Device (Covidien, Irvine, CA) and the Trevo Retriever (Stryker Neurovascular, Freemont, CA) are approved by the U.S. Food and Drug Administration for mechanical removal of clots. The Merci Retriever, a corkscrew shaped device, has been shown to provide a higher rate of good clinical outcome compared to historical controls, although the intracerebral hemorrhage rate was 7.8%, which is not different from IV rt-PA. Recent studies have demonstrated that the Solitaire FR and Trevo stent retrievers are superior to the Merci device. , In all cases patients eligible for intravenous rtPA should be given that treatment as rapidly as possible even if intra-arterial mechanical clot removal is being considered. Recent studies have found a clear outcome benefit of mechanical intra-arterial treatment for stroke; , , experience, rapidity of treatment and new devices have improved this technique to the point where its on-demand availability is rapidly becoming the standard of care for stroke centers. The 2015 AHA/ASA focused guideline update concludes that “Certain endovascular procedures have been demonstrated to provide clinical benefit in selected patients with acute ischemic stroke. Systems of care should be organized to facilitate the delivery of this care.” Patients for mechanical thrombectomy should have confirmed occlusions in the proximal anterior intracranial circulation and an absence of large ischemic-core lesions. As yet, however, there is no consensus as to the type of neuroimaging and the exact imaging criteria for patient eligibility, the rapidity of the assessment is critical, since delays lead to more infarcted brain. The accepted time window for treatment with mechanical thrombectomy is 6 hours from the onset of symptoms. For now, the most widely used effective treatment for ischemic stroke remains the administration of intravenous rtPA within 3 hours of stroke onset, or for up to 4.5 hours for certain patients. , This treatment is underutilized because of the short time window for safe administration of rtPA, the many contraindications to its use, and the frequent delay in presentation of patients to the hospital after the onset of symptoms. This problem can and is being addressed by community education about the signs of stroke through the FAST campaign (FACE, ARMS, SPEECH, TIME to call 911) and the need for emergency transport to an appropriate hospital with a stroke center. The guidelines for early management and treatment of stroke are frequently updated and should be consulted and examined for the latest recommendations.
Hypothermia
Deep hypothermia has long been used in neonatal heart surgery to provide protection against irreversible brain injury during circulatory arrest. It has also been used during the repair of giant aneurysms. However, there are numerous complications of profound hypothermia (27 °C or lower) that limit its usefulness ( Box 1.3 ). Profound hypothermia reduces cerebral metabolism to such an extent that the brain can survive relatively long periods without perfusion ( Box 1.4 ). Experimental studies indicate that moderate hypothermia has a protective effect without many of the complications of profound hypothermia, although myocardial depression has been documented. There are many in vitro and in vivo animal studies to support the use of moderate hypothermia to protect against ischemic damage. Indeed, moderate hypothermia has come into common use even though it has not been unequivocally shown to improve recovery in a major clinical trial. A European study published in 2002 indicates that mild hypothermia, target temperature 32° to 34 °C, after cardiac arrest improves neurologic outcome and survival 6 months after the arrest. However, recent larger studies have found no benefit to mild hypothermia compared to maintaining the temperature below 36 °C; avoiding hyperthermia appears to be important and may explain the benefit found in earlier studies. , The recommendation for hypothermia following cardiac arrest may soon be replaced by the avoidance of hyperthermia. However, with respect to hypothermia after stroke there is no Class I evidence of benefit; cooling to levels between 34° and 35 °C leads to fewer complications and slow rewarming is important to reduce deleterious effects. Mild hypothermia did not improve outcome from surgery for intracranial aneurysm surgery and a Cochrane systematic review did not find benefit or harm from hypothermia during acute stroke. , If hypothermia does demonstrate some benefit for certain types of stroke patients, its degree and duration as well as the rate of rewarming will need to be better determined.
Cardiovascular Complications
Myocardial depression
Dysrhythmia including ventricular fibrillation
Hypotension
Inadequate tissue perfusion
Ischemia
Coagulation
Thrombocytopenia
Fibrinolysis
Platelet dysfunction
Increased bleeding
Metabolism
Slowed metabolism of anesthetic agents
Prolonged neuromuscular blockade
Increased protein catabolism
Shivering
Increased oxygen consumption
Increased carbon dioxide production
Increased cardiac output
Arterial oxygen desaturation
Hemodynamic instability
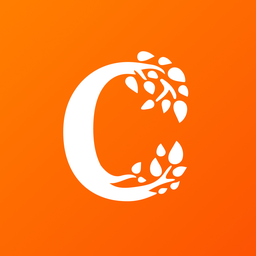
Full access? Get Clinical Tree
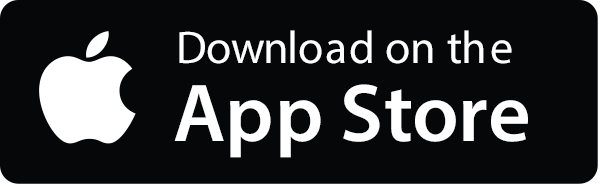
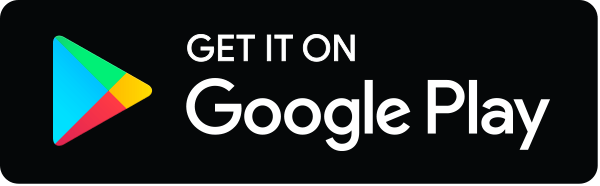
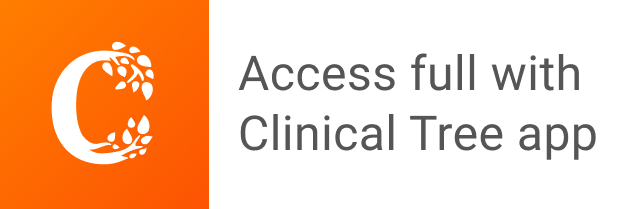