Fig. 9.1
(a) Diagrammatic axial section through the central sulcus at the level of the hand area of sensorimotor cortex, showing the earliest cortical dipoles activated after finger stimulation. Note the generator of the N/P20 in the postcentral gyrus , which is oriented parallel to the scalp so that the primary response reverses polarity from posterior–anterior, while the generator of P22 in the precentral gyrus is oriented perpendicular to the scalp so a surface electrode only sees the positive end of this dipole, which is activated slightly later. Responses seen at the scalp or cortical surface are a spatiotemporal summation of these two sources. (Adapted from Desmedt et al. [10]). (b) Photography of six contact strip electrode spanning the presumed central sulcus. (c) Responses to right (R) ulnar nerve stimulation recorded from cortical surface with four contract strip (traces 0–3) and scalp (C3′—Fz). Positivity is up; note N20 (at latency of ∼ 30 ms in this tall patient) in scalp recording and postcentral contact 0 with positivity at precentral contacts 1–3. Positivity shows longer latency in contact 1 due to contribution from second (P22) dipole in precentral gyrus
The polarity reversal of N/P20 is easy to visualize. Imagine a monopolar electrode, referenced to an inactive site, which is moved along the surface of the cortex perpendicular to the central sulcus at the time of the peak of N/P20. When the electrode is posterior to the generator (in the postcentral sulcus), a negativity will be recorded. As the electrode moves anteriorly, the negativity will reach a maximum and then drop to zero when the electrode is equidistant from the positive and negative ends of the dipole. As the electrode continues to move anteriorly, it will record the positivity, which will gradually decrease as the electrode is moved further forward away from the dipole area. If instead of a single electrode being moved, we now imagine a strip of electrodes with some contacts posterior and some anterior to the central sulcus, we see that the posterior electrodes will record a negativity at the same time as the anterior electrodes record a positivity. Paradoxically, an electrode contact directly over the dipole generator will record zero, even though it is closest to the active generator, because the contributions from the negative and positive poles of the dipole will cancel one another out.
The situation is slightly complicated by the fact that a second dipole, which is activated a few milliseconds later, is located in the precentral gyrus . This dipole, however, is oriented perpendicular to the cortical surface, and when activated causes a surface positivity and negativity that could only be recorded by a depth electrode underneath the pyramidal cell layer. This component is termed P22 and is recorded at the cortical surface only as positivity at a slightly longer latency than N20. Because an electrode at the cortical surface will record the sum of the activity of these two generators, the positivity over the precentral gyrus may appear to be at a slightly longer latency than the negativity seen posteriorly, depending on the exact orientation of the generators in relation to the location of the recording electrodes. With this minor caveat, the polarity reversal of the SSEP resulting from median nerve stimulation is easy to interpret, and will accurately define the location of the central sulcus. The polarity reversal can be easily recorded with a strip electrode, typically with four to eight contacts aligned at 1-cm intervals, or with a two-dimensional array or grid electrode (see Fig. 9.1b, c).
To record the polarity reversal , the electrode must be positioned so that it is over the hand area of the somatosensory cortex, with some contacts anterior and some posterior to the central sulcus. This may require adjustment of the electrode position to obtain optimal recordings. For example, if a negativity is recorded at all contacts, the entire electrode is probably posterior to the central sulcus and needs to be moved anteriorly. This happens more often than might be expected, particularly if preoperative functional imaging is not available. This is because the location of the central sulcus, which divides primary sensory from primary motor cortex, may vary by several centimeters from individual to individual with respect to external skull landmarks. This variability is why functional mapping is vitally important to avoid inadvertent resection of primary sensory and motor areas.
With a one-dimensional strip electrode, the waveforms can be simply displayed, one above the other, in the same order as the electrode contacts. This makes identification of the polarity reversal quite easy. With a grid electrode, the situation is more complicated, and ideally the waveforms should be displayed in a two-dimensional pattern corresponding to the arrangement of the contacts on the electrode. Alternatively, the distribution of electrical potentials at each time point can be displayed as a color map, in much the same fashion that temperature gradients are displayed in weather maps. For example, if negative potentials are displayed in different shades of blue and positive potentials in different shades of red, then the border between red and blue areas will define the central sulcus [12].
Finally, note that the SSEP polarity reversal can be recorded either from the surface of the cortex, as would be done prior to resection of a tumor in this region, or epidurally, for example during implantation of a motor cortex-stimulating electrode for control of chronic pain. In all cases, it is important to record at least one scalp channel of SSEP, in order to confirm the precise latency of N20, which may vary during a procedure, as it is significantly affected by peripheral temperature changes.
Because the SSEP signals recorded directly from the cortical surface are much larger than those recorded at the scalp, any anesthetic technique that is compatible with recording scalp SSEP will be suitable for cortical recordings. In fact, if SSEP recordings are the only mapping technique that will be utilized, neuromuscular blockade can be used, and will often improve the signal-to-noise ratio by eliminating the possibility of scalp EMG contamination. However, because motor mapping is usually done in the same context, neuromuscular blockade is generally contraindicated.
Location of the Primary Motor Cortex with Electrical Stimulation
The SSEP polarity reversal is often recorded first, as this provides a quick indication of the location of the central sulcus. Once the central sulcus is defined, and the presumed location of primary motor cortex is thus established, then the motor cortex is typically mapped with electrical stimulation. This complementary method confirms the results obtained with SSEP mapping and allows further delineation of the topographic organization of the motor cortex.
Until recently, motor mapping has been performed using essentially the same techniques pioneered by Penfield in the 1930s. The Ojemann OCS-2 Cortical Stimulator (Integra Life Sciences, Plainsboro, NJ) is used with a handheld electrode. Using bipolar electrical pulses of 1-ms duration at 60 Hz, and beginning at approximately 3-mA intensity, the electrode is placed on the cortical surface for about 1 s, and any movements on the contralateral side are noted. Yingling et al. [13] showed that the sensitivity of this mapping technique could be enhanced by simultaneous EMG recordings from multiple muscle groups contralateral to the side of stimulation (Fig. 9.2a). This method has several advantages; EMG activity can often be seen at much lower stimulation intensities than are necessary to produce overt movements, and the requirement to expose and observe the entire contralateral body is eliminated as it is relatively easy to scan multiple EMG channels for activity occurring at any location. Patients who are awake (i.e., with local anesthesia) may note “tugging” or other motor changes before visible motor activity occurs.
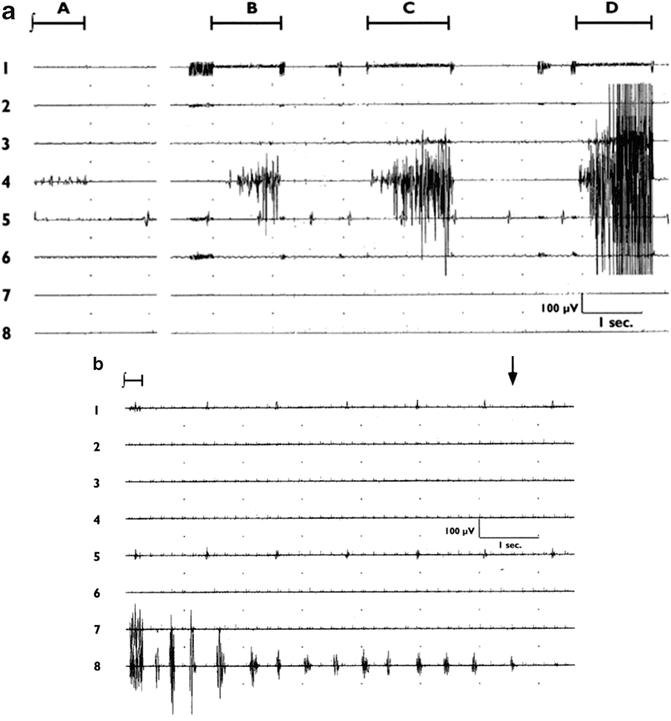
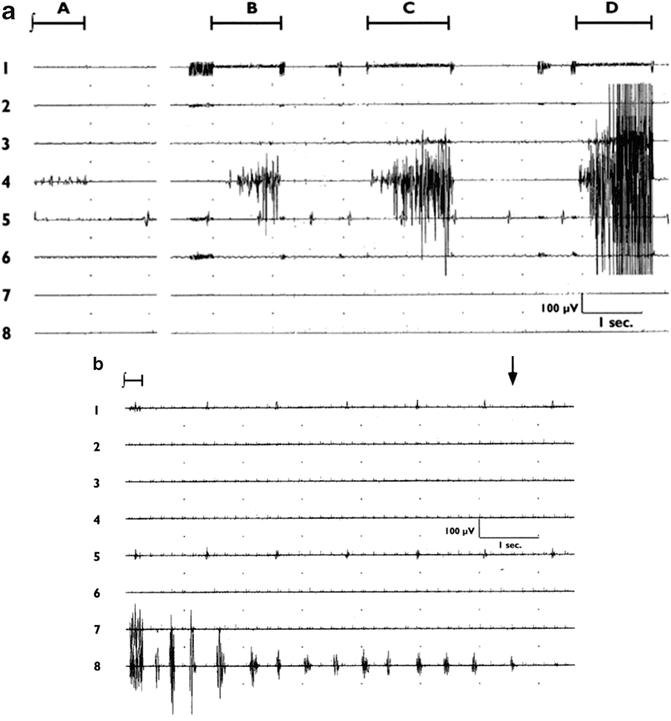
Fig. 9.2
(a) EMG responses to stimulation of the forearm area of cerebral cortex (time indicated by bars above traces) at 3.6 mA (A, B), 5 mA (C), and 6.2 mA (D). Traces (top to bottom) represent face, shoulder, upper arm, forearm, hand, upper leg, lower leg, and foot muscles. Overt movement was not evident except with the highest level of stimulation in trace D. (Reprinted with permission from Yingling et al. [13]). (b) Clonic seizure activity manifesting as rhythmic EMG activity in foot muscles after stimulation of the medial area of the sensorimotor cortex. (Stimulation began before the trace, as indicated by the bar at the top left). Activity was terminated by application of cold lactated Ringer’s solution to the cortical surface (time indicated by an arrow at the top right). (Reprinted with permission from Yingling et al. [13])
If no motor responses are obtained at an initial current setting of 3 mA, the current is increased, typically 1 mA at a time, and the mapping process is repeated until consistent responses are obtained and the organization of the cortical homunculus is confirmed. However, this method is not without its faults. In the Yingling et al. [13] study, 1-s stimulus trains elicited motor seizures in 24 % of the patients (Fig. 9.2b). While many of these were minor and self-terminating, they occasionally persisted or even increased in intensity or spread to other regions of the body, and had to be terminated by application of cold Ringer’s solution to the cortical surface [14]. A second problem with this technique is that it requires the active participation of the surgeon, and thus is a method for mapping, but does not permit continuous monitoring of corticospinal tract function during tumor resection. Transcranial MEPs (see Chap. 2, “Transcranial Motor-Evoked Potentials”) are often used for monitoring motor tract function, but transcranial stimulation may activate white matter tracts at a considerable depth below the surface of the cortex, and thus fail to detect motor pathway compromise at more superficial locations [15].
Years of experience with transcranial MEP recordings have demonstrated that this technique has an extremely low incidence of induced seizures [16]. This is presumably due to the brief duration of the stimulus trains employed, typically on the order of 10 ms, compared to the 1-s simulation used in the Penfield/Ojemann technique . This has led to the development of a new technique for cortical mapping under general anesthesia, using brief pulse trains similar to those employed with transcranial MEPs , but at a much lower intensity and applied directly to the cortical surface. While this technique had first been described by Taniguchi et al. [17], the first clinical series were published by Cedzich et al. [18] and Kombos et al. [19]. The combined data from these two studies show a success rate of 96 % in obtaining direct cortical MEP, with no seizures reported in either study.
We have had extensive experience using this technique in the San Francisco area during the past 8 years (Fig. 9.3). Typically, trains of 3–5 pulses, with 2-ms interstimulus intervals, are sufficient. Using 50-μs duration pulses, we typically obtain thresholds ranging from roughly 20–150 mA. Although this seems high when compared with thresholds obtained using the Ojemann or comparable stimulators , the total charge per pulse delivered to the brain is roughly equivalent. This is because the 50-μs duration pulses used in our train technique (due to limitations of the stimulator) are each 1/20 of the duration of the 1-ms pulses commonly employed in the 60-Hz technique. Because the total charge delivered to the brain is the product of current × time, the thresholds obtained with such brief pulses should be divided by 20 to compare them with the thresholds obtained using the traditional technique. Thus, these thresholds would be equivalent to 1–7.5 mA using a 1-ms pulse width.
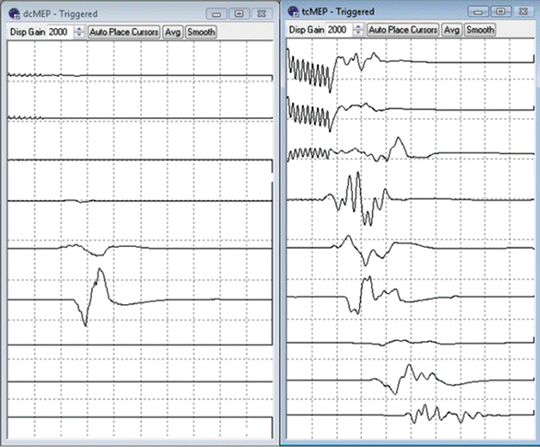
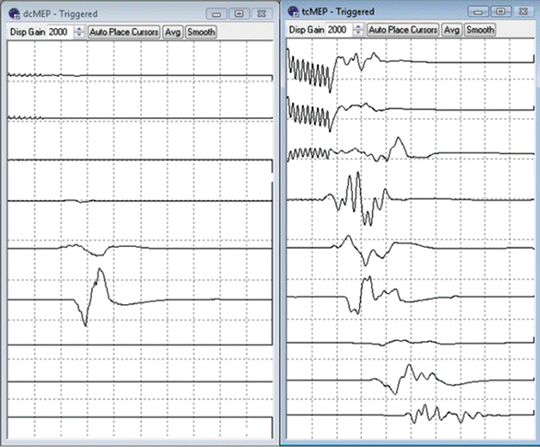
Fig. 9.3
MEP responses to direct cortical stimulation (left) and transcranial stimulation (right). Traces (top to bottom) orbicularis oris, trapezius, deltoid, biceps, flexor carpi ulnaris, abductor pollicis brevis, rectus femoris, gastrocnemius, and abductor hallucis. Note the focal nature of the responses to direct cortical stimulation (40 V), in contrast to responses seen in all channels following transcranial stimulation (300 V) due to spread of current through the scalp/skull and/or subcortical activation of the corticospinal tract
Note that the above comparisons are per pulse. With the traditional method, a 60-Hz pulse train is typically applied to the cortical surface for approximately 1 s, or 60 pulses total. (Even longer trains of 3–4 s duration are typically employed for language mapping.) In contrast, the direct cortical stimulation technique (dcMEP) delivers a total of 5 pulses or less, a full order of magnitude fewer than with the traditional technique. This is a probable reason why the dcMEP technique does not appear to induce seizure activity. In our recent series, of 118 patients in whom the dcMEP technique was used, 12 were also stimulated at 60 Hz with the Ojemann stimulator. Five of these 12 exhibited overt seizures or after discharges with 60-Hz stimulation, whereas none of the 118 patients had seizures following brief train stimulation (Yingling et al., unpublished data).
The lack of induced seizures and the minimal movement produced are the foundation for the second great advantage of the train technique over the traditional 60-Hz technique. Once the precentral gyrus has been mapped, a strip electrode can be left over the motor cortex and regularly stimulated while tumor resection proceeds. Unlike transcranial MEPs, which may produce overt movement requiring the surgeon to temporarily halt resection during MEP stimulation, the strip electrode can be repeatedly activated to provide continuous monitoring of corticospinal tract function during tumor resection. Thus, the dcMEP technique for motor mapping can also be used to provide continuous monitoring.
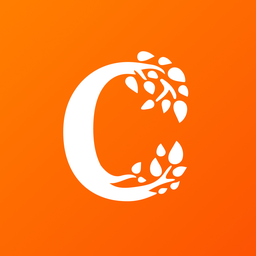
Full access? Get Clinical Tree
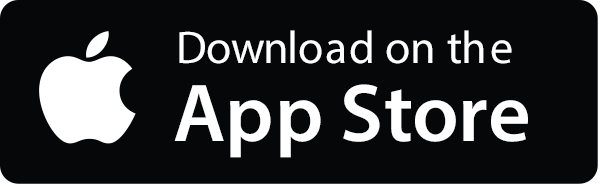
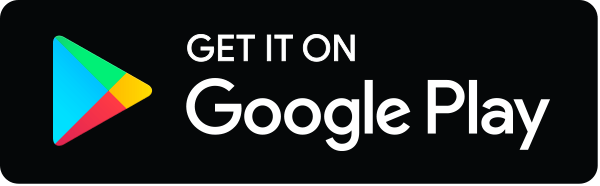