Muhammad Saad Yousuf1, Allan I. Basbaum2, & Theodore J. Price1 1 Center for Advanced Pain Studies, School of Behavioral and Brain Sciences, University of Texas at Dallas, Dallas, Texas, USA 2 Department of Anatomy, University of California at San Francisco. San Francisco, California, USA The ability to experience pain is essential for survival and wellbeing and the pathological consequences of the inability to experience pain are particularly well‐illustrated by the extensive injuries experienced by children with congenital insensitivity to pain [1]. On the other hand, the need for better pain relief therapeutics is urgent and, in particular for chronic pain, has been a contributing factor to the international opioid crisis [2]. The neural basis of pain processing, including afferent fibers (nociceptors) that respond to injury, and the circuits engaged by these afferents, not only generate reflex withdrawal to injury, but also provide a protective function following tissue or nerve injury. These pain sensitizing mechanisms following an injury promote tissue repair and enhance evolutionary fitness of an organism [3, 4]. In these situations, neurons in the pain processing circuitry become sensitized such that normally innocuous stimuli are perceived as painful (allodynia), and normally noxious stimuli are perceived as even more painful (hyperalgesia). The sensitization process is presumably an adaptive response, in that it promotes protective guarding of an injured area. In some cases, however, sensitization can be long‐lasting, leading to the establishment of chronic pain syndromes. In this situation, the sensitization outlives its usefulness, persisting well after the acute injury has resolved. In these pathological, often debilitating conditions, aberrant plasticity in pain circuitry establishes a maladaptive condition in which pain no longer serves as an acute warning system. The ability to prevent or treat such conditions is critically dependent upon a comprehensive understanding of the basic mechanisms through which pain signals are generated by nociceptors, how this information is transmitted to the central nervous system (CNS) as well as how the CNS modulates incoming nociceptive information. In this chapter, we focus on the molecules and cell types that underlie normal pain sensation, with specific emphasis on the nociceptor and on second order neurons in the spinal cord. We also discuss how these circuits are altered following tissue or nerve injury and in persistent pain states. The detection of somatosensory stimuli is initiated by primary sensory neurons that have their cell bodies in the trigeminal (TG) and dorsal root ganglia (DRG). These pseudo‐unipolar neurons extend an efferent branch that innervates peripheral target tissues, and a central afferent branch that targets the spinal cord dorsal horn or medullary nucleus caudalis (for trigeminal afferents). Primary afferents that innervate somatic tissue are traditionally classified into three categories: Aβ, Aδ and C fibers, based on axon diameter, degree of myelination, and conduction velocity. These physiological differences are associated with distinct functional contributions to somatosensation. The largest diameter cell bodies give rise to myelinated Aβ fibers that rapidly conduct nerve impulses and detect innocuous mechanical stimulation. In contrast, noxious thermal, mechanical, and chemical stimuli are detected by medium diameter, thinly myelinated Aδ fibers, and by small diameter, unmyelinated C fibers. These latter two groups constitute the nociceptors and represent a dedicated system for the detection of stimuli capable of causing tissue damage, as they are only excited when stimulus intensities reach the noxious range [5]. The Aδ nociceptors mediate the fast, pricking sensation of “first pain,” and the C fibers convey information leading to the sustained, often burning quality of “second pain”. Another lesser‐known class of C fibers are C‐low threshold mechanoreceptors (C‐LTMRs) that innervate hairy skin and are typically associated with affective aspects of touch. The contribution of C‐LTMRs to pain modulation has only recently come to light [6, 7]. Electrophysiology studies have identified two main classes of Aδ nociceptor. The first class is readily activated by intense mechanical stimulation. These neurons are relatively unresponsive to short duration, noxious heat stimulation, but respond more robustly to extended periods of heat stimulation [8]. The second class is insensitive to mechanical stimulation but is robustly activated by heat [8]. The majority of C‐fiber nociceptors show polymodal response properties. They are activated by multiple modalities of painful stimuli, including thermal, chemical and mechanical. Although rarer, modality‐specific (e.g. exclusively heat‐responsive) C fibers also exist. These molecularly defined C‐fiber subtypes make functionally distinct contributions to the detection of noxious stimuli of different modalities [9]. Recent advances in RNA sequencing‐based transcriptomics, largely in mice, have further delineated sensory neurons into at least 11 different subtypes based on their unique RNA expression profiles [10] (Figure 3.1). These neurons are further grouped into 4 main categories consisting of large diameter myelinated afferents that express the 200kD neurofilament protein (NEFH), peptidergic afferents that express neuropeptides [including calcitonin gene‐related peptide (CGRP)], non‐peptidergic afferents that lack neuropeptides and bind the isolectin B4 (IB4) and tyrosine hydroxylase‐expressing C‐LTMRs. Additional characteristics of these sensory neurons are presented in Figure 3.1. Single cell sequencing and other cellular characterization methods are now being used in non‐human primate [11] and human DRG studies [12]. Importantly, molecular identification of sensory neuron subtypes in mice has enabled identification of genetic tools to elucidate cell‐type specific functions in the behaving animal. These experimental tools should provide powerful insights into conserved, and divergent, functions of genes in nociceptors, across species and should also improve target identification and validation for clinical translation. Figure 3.1 Primary sensory neuron characteristics. Primary sensory neurons are categorized based on their conduction velocity, degree of myelination and thickness of their axons and cell bodies. While the axons of Aα/β large‐diameter and Aδ medium‐diameter neurons are thickly and thinly myelinated, respectively, C fibres are unmyelinated and supported by Schwann cells organized in Remak bundles. Recent single cell RNA sequencing by Usoskin, Furlan [10] has revealed 11 sensory neuronal subtypes based on their molecular composition. Aα/β fibres are classified as low‐threshold mechanoreceptors (LTMRs) and proprioceptors. Nociceptors (Aδ and C fibres) are further grouped by whether they produce neuropeptides (peptidergic), like calcitonin‐gene related peptide (CGRP), or not (non‐peptidergic). C‐low threshold mechanoreceptors (C‐LTMRs), a special class of C fibres, are involved in non‐noxious, affective touch and are characterized by their expression of tyrosine hydroxylase. Myelinated fibres (Aα/β and Aδ) express the heavy neurofilament polypeptide (NEFH). Nociceptors (Aδ and C) are characterized by the presence of voltage‐gated sodium channels 1.8 and 1.9 (Nav1.8/1.9). Other molecular markers are summarized. The peripheral terminal of the nociceptor is specialized to detect and transduce noxious stimuli. This process depends on the presence of specific ion channels and receptors at the peripheral terminal (Figure 3.2). Among these are the acid‐sensing ion channels (ASICs), purinergic P2X receptors, voltage‐gated sodium, calcium and potassium channels and the transient receptor potential (TRP) family of ion channels [8, 13]. Notably, many of these molecules are uniquely or preferentially expressed in nociceptors, compared to other parts of the nervous system. The activation thresholds of several peripheral receptors closely match the psychophysical demarcation between the perception of innocuous and noxious thermal stimuli. For example, the heat pain threshold in humans, which rests around 43°C, matches the activation threshold for the sensory ion channel, TRPV1, and mice lacking TRPV1 exhibit deficits in cellular and behavioral responses to noxious heat [13]. In addition, several other receptors contribute to the detection of noxious thermal stimuli, including TRPV2, TRPV3, TRPV4 and TRPM2 ion channels [13]. Cold sensitive neurons are a mix of low‐threshold and high‐threshold thermoreceptors, each of which respond to a gradient of cool temperatures from mild to extreme. Low‐threshold cold‐sensitive neurons are tonically active and respond to cooler temperatures by increasing their firing frequency. On the contrary, high threshold cold‐sensitive neurons are only active in the noxious temperature range, below about 20°C. At subzero temperatures all nociceptors, even cold‐insensitive ones, fire action potentials, possibly due to tissue damage. TRPM8, an ion channel sensitive to menthol, is activated by temperatures below 20°C, and mice lacking this receptor show a drastic reduction in their responses to a range of cool and cold temperatures [14]. More importantly, TRPM8 knockout animals still have preserved sensitivity to noxious cold stimuli suggesting that another cold‐sensitive channel may also contribute to discriminating painful cold stimuli. As such, TRPA1 is activated at temperatures below 10°C in recombinant assays and may further encode noxious cold sensation [14]. However, there remains a debate as to whether TRPA1, which responds to a host of irritants, is a genuine cold receptor. Several candidate receptors have been proposed to underlie the transduction of mechanical stimuli. Rapidly adapting neurons have a low threshold to mechanical stimulation and are involved in innocuous touch and proprioception. Mechano‐sensitive nociceptors, however, include rapidly, moderately and slowly adapting currents with high activation thresholds, allowing them to encode noxious stimuli. Various members of the degenerin/ epithelial Na+ channel (DEG/ENaC) families, the TRP family (e.g. TRPV2, TRPV4 and TRPA1), and the Piezo family (Piezo1 and Piezo2) have been implicated in mechanonociception. Piezos are the most important family of mechanically‐gated channels in the mammalian genome. Piezo2 is predominantly expressed in the sensory nervous system and is gated by mechanical stimulation following gentle touch [15]. Its contribution to mechanical allodynia has only recently been described. Notably, individuals with loss‐of‐function mutations in the Piezo2 channel fail to develop mechanical allodynia following skin inflammation; however, their sensitivity to noxious mechanical stimulation is preserved [16, 17]. TACAN was recently identified as a mechanically‐activated ion channel that is encoded by the Tmem120A gene [18]. This mechanically‐activated channel is expressed in nociceptors and may be involved in mechanical hyperalgesia [18, 19]. Figure 3.2 Primary afferent terminals. The sensory afferent terminal preferentially responds to a plethora of stimuli using a panoply of receptors and ion channels. Heat, cold and chemical toxins are encoded by Ca2+‐permeable transient receptor potential (TRP) channels such as TRPV1, TRPM8, and TRPA1. Low pH (pH<6) is also known to activate acid‐sensing ion channels (ASICs). Purinergic receptors, particularly P2X receptors, respond to extracellular ATP usually released in response to an inflammatory insult. Recent characterization of pressure‐sensitive receptors has identified Piezo2 and TACAN channels as selective mechanosensors important for the development of mechanical allodynia and hyperalgesia. More recently, a class of Schwann cells that transduce tactile nociception were discovered to ensheathe free nerve endings [86]. Tissue injury and inflammation causes the release of various factors that enhance the activity of nerve fibres and promote sensitization. These factors are summarized in the figure. Noxious chemical stimuli activate a range of receptors found in nociceptor terminals. Among these are the ASICs and ATP‐responsive purinergic receptors, which may be especially relevant in the setting of tissue injury (where pH changes and ATP release are common). Some TRP channels (e.g. TRPV1) are also regulated by pH and many are targets of plant‐derived irritants, including capsaicin (TRPV1), menthol (TRPM8) and the pungent ingredients in mustard and garlic plants (TRPA1). TRPA1 also responds to a host of environmental irritants [20]. Undoubtedly there are endogenous chemical mediators that activate the different TRP channel subtypes. These mediators may be especially critical in the setting of injury to visceral tissue, the afferent innervation of which is not accessible to exogenous chemical or intense thermal stimuli. Finally, nociceptors have recently been implicated in host defense. These neurons respond directly to bacterial infection through mechanisms that can be mediated directly by bacterial factors that form pores in nociceptor membranes or through direct actions of bacterial mediators on host receptors [21, 22]. An excellent example of the latter is sulfolipid‐1 a product of the tuberculosis pathogen that evokes cough through a direct action on nociceptors [23]. Nociceptors also respond to type I interferons, which are rapidly produced in response to viral infection [24]. Moreover, the activation of nociceptor nerve endings leads to the release of CGRP and substance P (SP) that then promote neurogenic inflammation. CGRP functions as a vasodilator and SP causes vascular leakiness both of which ultimately promote immune infiltration. Therefore, nociceptors are clearly important contributors in the response to infection. Nociceptors express a panoply of voltage‐gated ion channel subtypes. Among these are the sensory neuron‐specific sodium channels Nav1.8 and 1.9, which, along with the more broadly expressed sodium channel Nav1.7, contribute to the generation and transduction of action potentials in nociceptors [1, 25]. A pivotal role for Nav1.7 in nociception has been demonstrated by the report that loss‐of‐function mutations of this channel in humans lead to the inability to detect painful stimuli, while gain‐of‐function mutations lead to disorders characterized by intense burning pain [1, 25]. Mice lacking Nav1.7 fail to develop pain hypersensitivity to noxious heat, mechanical and chemical (e.g. formalin) stimuli [1]. Furthermore, these animals are unaffected by complete Freund’s adjuvant treatment, suggesting that inflammatory pain relies on Nav1.7‐mediated spike generation. Interestingly, deleting Nav1.7 in Nav1.8‐positive sensory neurons prevents the development of mechanical allodynia but not heat hypersensitivity [1]. Particularly provocative is the recent report that the loss of function after NaV1.7 deletion occurs concomitantly with a naloxone‐reversible increase in enkephalin‐mediated inhibitory controls in the dorsal horn [26, 27]. With over 20 different K+ channels coupled with a plethora of auxiliary subunits, K+ channels have versatile electrophysiology properties that are integral to the falling phase of the action potential [28]. Following nerve injury, K+ currents are typically attenuated, which accommodate persistent action potential generation. The KCNQ type of potassium channel produces M‐currents that determine the repolarization time of nociceptors [28]. Importantly, many inflammatory mediators, including interleukin 1β, can modulate these K+ currents in nociceptors resulting in an increase overall excitability and neurotransmitter release [28]. Once an action potential invades the central terminal of a nociceptor, neurotransmitter release is evoked via the activation of N‐, P/Q‐ and T‐type voltage‐gated calcium channels. Although glutamate is the predominant, if not the obligatory, excitatory neurotransmitter in all nociceptors, many nociceptors co‐release release substance P (SP) and CGRP [8]. Receptors for these neurotransmitters, including N‐methyl‐D‐aspartic acid (NMDA) and α‐amino‐3‐hydroxy‐5‐methyl‐4‐isoxazolepropionic acid (AMPA) receptors for glutamate, neurokinin 1 receptors for SP and CGRP receptors, are located in appropriate regions of the spinal cord dorsal horn, and mediate the postsynaptic response to primary afferent activation [29]. Nociceptors not only transmit pain messages, centrally to the spinal cord, but also release a variety of molecules from their peripheral terminals. These molecules (e.g. the neuropeptides SP and CGRP) influence the local tissue environment by acting on blood vessels and other cells to cause vasodilatation and plasma extravasation, key features of neurogenic inflammation (Figure 3.2). Neurogenic inflammation alters the extracellular milieu of the peripheral terminals of nociceptors, which can sensitize the nociceptor to subsequent stimulation. It is now understood that these sensory neuropeptides are also involved in immune responses to infection [30, 31] and in immunological tissue homeostasis [32] (Figure 3.2). In addition to detecting noxious insults, nociceptors likely play a key homeostatic role that contributes to overall fitness. The biochemical complexity of nociceptor subtypes is paralleled by their distinct peripheral innervation patterns. For example, some markers delineate populations of nociceptors whose peripheral innervation is restricted to particular tissues. Thus, nociceptors that express the Mrgprd subtype of G‐protein coupled receptor innervate skin, but not visceral organs [33, 34]. Interestingly, these Mrgprd+ neurons in the trigeminal ganglion innervate the meninges of the brain, a specialized tissue that protects the brain and forms the blood brain barrier [35]. The central branches of primary afferents terminate in the dorsal horn of the spinal cord, which is classically divided into six parallel laminae, based on cytoarchitectural grounds [29]. Neurons in lamina III and IV are innervated by myelinated fibers that respond to innocuous touch (Figure 3.3). In contrast, neurons in laminae I, II and V receive inputs from nociceptive afferents and are therefore important relays in the transmission of pain‐related information, both locally and via projection neurons of laminae I and V that target the brain [29]. Lamina VI neurons receive input from muscle. Recent single‐cell RNA sequencing experiments have identified 15 inhibitory and 15 excitatory neuronal subtypes in the dorsal horn, revealing a diverse, heterogeneous population of spinal neurons [36]. The remarkable stratification of spinal cord inputs is further demonstrated by the distinct projection patterns of Aδ and C‐fiber nociceptors (Figure 3.3). Lamina I spinal cord neurons are innervated by both Aδ and C fibers. Consistent with this input, the majority of neurons in lamina I are selectively activated by noxious stimuli and are thus referred to as nociceptive‐specific neurons. Lamina I also contains so‐called wide dynamic range (WDR) neurons, which receive convergent monosynaptic input from nociceptors and polysynaptic input from non‐nociceptive fibers. Neurons that appear to encode selectively innocuous sensations such as cooling, itch and sensual touch are also found in lamina I [37, 38]. Although most lamina I neurons are interneurons that are engaged in local dorsal horn circuits, a small but critical number (∼10%) are projection neurons that directly access pain processing centers in the brain [29].
Chapter 3
Basic mechanisms and pathophysiology
Introduction
Primary afferent neurons
Nociceptor subtypes
Nociceptors and noxious stimulus detection
Conduction of nociceptive signals
Organization of the “pain system”
The afferent terminal
Central projections of nociceptors
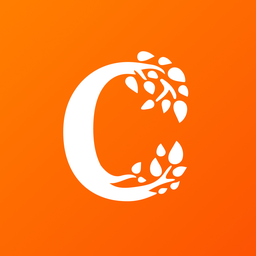
Full access? Get Clinical Tree
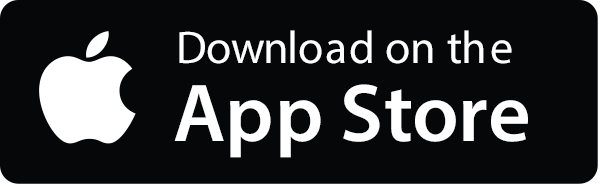
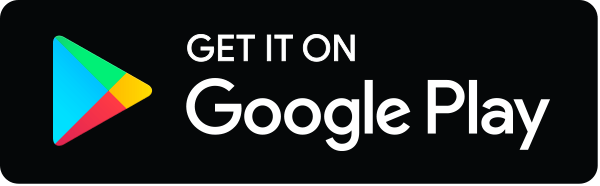