Key Points
- 1.
An ultrasound beam is a continuous or intermittent train of sound waves emitted by a transducer or wave generator that is composed of density or pressure. Ultrasound waves are characterized by their wavelength, frequency, and velocity.
- 2.
Doppler frequency shift analysis can be used to obtain blood-flow velocity, direction, and acceleration of red blood cells, in which the magnitude and direction of the frequency shift are related to the velocity and direction of the moving target. These velocity flow measurements may be used to determine gradients and blood-flow volumes.
- 3.
Axial resolution is the minimum separation between two interfaces located in a direction parallel to the ultrasound beam, enabling them to be imaged as two different interfaces. Lateral resolution is the minimum separation of two interfaces aligned along a direction perpendicular to the beam. Elevational resolution refers to the ability to determine differences in the thickness of the imaging plane.
- 4.
Absolute contraindications to transesophageal echocardiography in intubated patients include esophageal stricture, diverticula, tumor, recent suture lines, and known esophageal interruption. Relative contraindications include symptomatic hiatal hernia, esophagitis, coagulopathy, esophageal varices, and unexplained upper gastrointestinal bleeding.
- 5.
Horizontal imaging planes are obtained by moving the transesophageal echocardiography probe up and down (upper esophageal: 20–25 cm; midesophageal: 30–40 cm; transgastric: 40–45 cm; deep transgastric: 45–50 cm). Multiplane probes may further facilitate the interrogation of complex anatomic structures by allowing up to 180 degrees of axial rotation of the imaging plane without manual probe manipulation.
- 6.
Aortic stenosis may be evaluated by planimetry, transaortic gradients, or the continuity equation. The use of planimetry is usually limited by the presence of aortic valvular calcifications. Peak, as well as mean, gradients may be measured using continuous-wave Doppler over the aortic valve in either the deep transgastric or transgastric long-axis view. The continuity equation uses measurement of flow through the left ventricular outflow tract and the aortic valve to determine aortic valve area.
- 7.
Quantification of aortic regurgitation is usually based on the analysis of color-flow Doppler patterns in the left ventricular outflow tract during diastole. The most reliable measurements are the vena contracta width and the ratio of proximal jet width to the width of the left ventricular outflow tract.
- 8.
Mitral stenosis may be evaluated by planimetry of the valve in the transgastric basal short-axis view. Transmitral Doppler spectral analysis may be used to calculate mean transmitral gradient and mitral valve area using the pressure half-time measurement of the E wave.
- 9.
Mitral regurgitation may be quantified by the analysis of color-flow Doppler spectra in the left atrium during ventricular systole. The severity of regurgitation may be further quantified using an analysis of pulmonary venous blood-flow velocities and measurements of regurgitant orifice areas using proximal isovelocity surface area. The vena contracta width measurement is easily deployed and is a reproducible technique for assessing mitral regurgitation.
Few areas in cardiac anesthesia have developed as rapidly as the field of intraoperative echocardiography. In the early 1980s, when transesophageal echocardiography (TEE) was first used in the surgical unit, its primary application was the assessment of global and regional left ventricular (LV) function. Since that time, there have been numerous technical advances: biplane and multiplane probes; multifrequency probes; enhanced scanning resolution; color-flow Doppler (CFD), pulsed-wave Doppler (PWD), and continuous-wave Doppler (CWD); automatic edge detection; Doppler tissue imaging (DTI); three-dimensional (3D) reconstruction; and digital image processing. With these advances, the number of clinical applications of TEE has significantly increased. The common applications of TEE include (1) assessment of valvular anatomy and function, (2) evaluation of the thoracic aorta, (3) detection of intracardiac defects, (4) detection of intracardiac masses, (5) evaluation of pericardial effusions, (6) detection of intracardiac air and clots, and (7) assessment of biventricular systolic and diastolic function. In many of these evaluations, TEE is able to provide unique and critical information that was not previously available in the surgical unit ( Box 11.1 ).

Assessment of valvular anatomy and function
Evaluation of the thoracic aorta
Detection of intracardiac defects
Evaluation of pericardial effusions
Detection of intracardiac air, clots, or masses
Assessment of biventricular systolic and diastolic function
Evaluation of myocardial ischemia
Basic Concepts
Properties of Ultrasound
In echocardiography, the heart and great vessels are insonated with ultrasound, which is sound above the human audible range. The ultrasound is sent into the thoracic cavity and is partially reflected by the cardiac structures. From these reflections, distance, velocity, and density of objects within the chest are derived.
An ultrasound beam is a continuous or intermittent train of sound waves emitted by a transducer or wave generator. It comprises density or pressure waves and can exist in any medium with the exception of a vacuum. Ultrasound waves are characterized by their wavelength, frequency, and velocity. Wavelength is the distance between the two nearest points of equal pressure or density in an ultrasound beam, and velocity is the speed at which the waves propagate through a medium. As the waves travel past any fixed point in an ultrasound beam, the pressure cycles regularly and continuously between a high and low value. The number of cycles per second (measured in hertz [Hz]) is called the frequency of the wave. Ultrasound is sound with frequencies above 20,000 Hz, which is the upper limit of the human audible range. The relationship among the frequency (f), wavelength (λ), and velocity (v) of a sound wave is defined by the following formula:
v = f × λ
Basic Concepts
Properties of Ultrasound
In echocardiography, the heart and great vessels are insonated with ultrasound, which is sound above the human audible range. The ultrasound is sent into the thoracic cavity and is partially reflected by the cardiac structures. From these reflections, distance, velocity, and density of objects within the chest are derived.
An ultrasound beam is a continuous or intermittent train of sound waves emitted by a transducer or wave generator. It comprises density or pressure waves and can exist in any medium with the exception of a vacuum. Ultrasound waves are characterized by their wavelength, frequency, and velocity. Wavelength is the distance between the two nearest points of equal pressure or density in an ultrasound beam, and velocity is the speed at which the waves propagate through a medium. As the waves travel past any fixed point in an ultrasound beam, the pressure cycles regularly and continuously between a high and low value. The number of cycles per second (measured in hertz [Hz]) is called the frequency of the wave. Ultrasound is sound with frequencies above 20,000 Hz, which is the upper limit of the human audible range. The relationship among the frequency (f), wavelength (λ), and velocity (v) of a sound wave is defined by the following formula:
Piezoelectric crystals convert between ultrasound and electrical signals. When presented with a high-frequency electrical signal, these crystals produce ultrasound energy; conversely, when they are presented with an ultrasonic vibration, they produce an alternating current electrical signal. Commonly, a short ultrasound signal is emitted from the piezoelectric crystal, which is directed toward the areas to be imaged. After ultrasound wave formation, the crystal “listens” for the returning echoes for a given period and then pauses before repeating this cycle. This cycle length is known as the pulse repetition frequency (PRF). This cycle length must be long enough to provide enough time for a signal to travel to and return from a given object of interest. When reflected ultrasound waves return to these piezoelectric crystals, they are converted into electrical signals, which may be appropriately processed and displayed. Electronic circuits measure the time delay between the emitted and received echoes. Since the speed of ultrasound through tissue is constant, this time delay may be converted into the precise distance between the transducer and tissue. The amplitude or strength of the returning ultrasound signal provides information about the characteristics of the insonated tissue.
Imaging Techniques
M Mode
The most basic form of ultrasound imaging is M-mode echocardiography. In this mode, the density and position of all tissues in the path of a narrow ultrasound beam (ie, along a single line) are displayed as a scroll on a video screen. The scrolling produces an updated, continuously changing time plot of the studied tissue section several seconds in duration. Because this is a timed motion display (normal cardiac tissue is always in motion), it is called M mode . Because only a very limited part of the heart is being observed at any one time and because the image requires considerable interpretation, M mode is not currently used as a primary imaging technique. M mode is, however, useful for the precise timing of events within the cardiac cycle and is often used in combination with CFD for the timing of abnormal flows
Two-Dimensional Mode
By rapid, repetitive scanning along many different radii within an area in the shape of a fan (sector), echocardiography generates a two-dimensional (2D) image of a section of the heart. This image, which resembles an anatomic section, can be more easily interpreted than an M-mode display. Information on structures and motion in the plane of a 2D scan is updated 20 to 40 times per second. This repetitive update produces a live (real-time) image of the heart. Scanning 2D echocardiographic devices usually image the heart using an electronically steered ultrasound beam (phased-array transducer).
Doppler Techniques
Most modern echocardiographic scanners combine Doppler capabilities with their 2D imaging capabilities. After the desired view of the heart has been obtained with 2D echocardiography, the Doppler beam, represented by a cursor, is superimposed on the 2D image. The operator positions the cursor as parallel as possible to the assumed direction of blood flow and then empirically adjusts the direction of the beam to optimize the audio and visual representations of the reflected Doppler signal. At the present time, Doppler technology can be used in at least four different ways to measure blood velocities: pulsed, high-repetition frequency, continuous-wave, and color-flow.
Color-Flow Doppler
Advances in electronics and computer technology have allowed the development of CFD ultrasound scanners capable of displaying real-time blood flow within the heart as colors while also showing 2D images in black and white. In addition to showing the location, direction, and velocity of cardiac blood flow, the images produced by these devices allow the estimation of flow acceleration and differentiation of laminar from turbulent blood flow. CFD echocardiography is based on the principle of multigated PWD in which blood-flow velocities are sampled at many locations along many lines covering the entire imaging sector. At the same time, the sector is also scanned to generate a 2D image.
A location in the heart where the scanner has detected flow toward the transducer (the top of the image sector) is assigned the color red. Flow away from the direction of the top is assigned the color blue. This color assignment is arbitrary and determined by the equipment’s manufacturer and the user’s color mapping. In the most common color-flow coding scheme, the faster the velocity (up to a limit), the more intense the color. Flow velocities that change by more than a preset value within a brief time interval (flow variance) may have an additional hue added to either the red or the blue. Both rapidly accelerating laminar flow (change in flow speed) and turbulent flow (change in flow direction) satisfy the criteria for rapid changes in velocity. In summary, the brightness of the red or blue colors at any location and time is usually proportional to the corresponding flow velocity, whereas the hue is proportional to the temporal rate of change of the velocity.
Equipment
All TEE probes share several common features. All of the currently available probes use a multifrequency transducer that is mounted on the tip of a gastroscope housing. The majority of the echocardiographic examination is performed using ultrasound between 3.5 and 7 MHz. The tip can be directed by the adjustment of knobs placed at the proximal handle. There are two knobs in most probes for adults; one allows anterior and posterior movement, and the other permits side-to-side motion. Multiplane probes also include a control to rotate the echocardiographic array mechanically from 0 to 180 degrees. Thus in combination with the ability to advance and withdraw the probe and to rotate it, many echocardiographic windows are possible. Another feature common to most probes is the inclusion of a temperature sensor to warn of possible heat injury from the transducer to the esophagus.
Currently, most adult echocardiographic probes are multiplane (variable orientation of the scanning plane), whereas pediatric probes are either multiplane or biplane (transverse and longitudinal orientation, parallel to the shaft). The adult probes usually have a shaft length of 100 cm and are between 9 and 12 mm in diameter. The tips of the probes vary slightly in shape and size but are generally 1 to 2 mm wider than the shaft. The size of these probes requires the patient to weigh at least 20 kg. Depending on the manufacturer, the adult probes contain between 32 and 64 elements per scanning orientation. In general, the image quality is directly related to the number of elements used. The pediatric probes are mounted on a narrower, shorter shaft with smaller transducers. These probes may be used in patients weighing as little as 1 kg.
An important feature that is often available is the ability to alter the scanning frequency. A lower frequency, such as 3.5 MHz, has greater penetration and is more suited for the transgastric (TG) view. It also increases the Doppler velocity limits. Conversely, the higher frequencies yield better resolution for detailed imaging. One of the limitations of TEE is that structures very close to the probe are seen only in a very narrow sector. Newer probes may also allow a broader near-field view. Finally, newer probes possess the ability to scan simultaneously in more than one plane. Nonmechanical matrix array probes use a diced crystal stack that can scan both laterally and elevationally. This 2D array can create a 3D ultrasound image and can also create simultaneous intersecting 2D images.
Complications
Complications resulting from intraoperative TEE can be separated into two groups: injury from direct trauma to the airway and esophagus and indirect effects of TEE ( Box 11.2 ). In the first group, potential complications include esophageal bleeding, burning, tearing, dysphagia, and laryngeal discomfort. Many of these complications could result from pressure exerted by the tip of the probe on the esophagus and the airway. Although maximum flexion of the probe will not result in pressure above 17 mm Hg in most patients, occasionally, even in the absence of esophageal disease, pressures greater than 60 mm Hg will result.
Injury from direct trauma to the airway and esophagus
- •
Esophageal bleeding, burning, tearing
- •
Dysphagia
- •
Laryngeal discomfort
- •
Bacteremia
- •
Vocal cord paralysis
- •
Indirect effects
- •
Hemodynamic and pulmonary effects of airway manipulation
- •
Distraction from patient care
Further confirmation of the low incidence of esophageal injury from TEE is apparent in the few case reports of complications. In the literature there are only a few reports of a fatal esophageal perforation and benign Mallory-Weiss tear after intraoperative TEE. Therefore, if resistance is met while advancing the probe, then the procedure should be aborted to avoid potentially lethal complications.
The second group of complications that results from TEE includes hemodynamic and pulmonary effects of airway manipulation and, particularly for new TEE operators, distraction from patient care. Fortunately, in the anesthetized patient, hemodynamic consequences to esophageal placement of the probe are rare, and no studies specifically address this issue. More important for the anesthesiologist are the problems of distraction from patient care. Although these reports have not appeared in the literature, the authors have heard of several endotracheal tube disconnections that went unnoticed to the point of desaturation during TEE examination. Additionally, instances during which severe hemodynamic abnormalities have been missed because of a fascination with the images or the controls of the echocardiograph machine have been reported. Clearly, new echocardiographic operators should enlist the assistance of an associate to watch the patient during the echocardiographic examination. This second anesthesiologist will become unnecessary after sufficient experience is gained. Ensuring that all respiratory and hemodynamic alarms are activated during the echocardiographic examination is also important.
Safety Guidelines and Contraindications
To ensure the continued safety of TEE, the following recommendations are made. The probe should be inspected before each insertion for cleanliness and structural integrity. If possible, the electrical isolation should also be checked. The probe should be inserted gently; if resistance is met, then the procedure should be aborted. Minimal transducer energy should be used, and the image should be frozen when not in use. Finally, when not imaging, the probe should be left in the neutral, unlocked position to avoid prolonged pressure on the esophageal mucosa.
Absolute contraindications to TEE in intubated patients include esophageal stricture, diverticula, tumor, recent suture lines, and known esophageal interruption. Relative contraindications include symptomatic hiatal hernia, esophagitis, coagulopathy, esophageal varices, and unexplained upper gastrointestinal bleeding. Notably, despite these relative contraindications, TEE has been used in patients undergoing hepatic transplantation without reported sequelae.
Technique of Probe Passage
The passage of a TEE probe through the oral and pharyngeal cavities in anesthetized patients may be challenging at times. The usual technique is to place the well-lubricated probe in the posterior portion of the oropharynx with the transducer element pointing inferiorly and anteriorly. The remainder of the probe may be stabilized by looping the controls and the proximal portion of the probe over the operator’s neck and shoulder. The operator’s left hand then elevates the mandible by inserting the thumb behind the teeth, grasping the submandibular region with the fingers, and then gently lifting. The probe is then advanced against a slight but even resistance, until a loss of resistance is detected as the tip of the probe passes the inferior constrictor muscle of the pharynx, which usually occurs 10 cm past the lips in neonates to 20 cm past the lips in adults. Further manipulation of the probe is performed under echocardiographic guidance.
Difficult TEE probe insertion may be caused by the probe tip abutting the pyriform sinuses, vallecula, posterior tongue, or an esophageal diverticulum. Overinflation of the endotracheal tube cuff could also obstruct passage of the probe. Maneuvers that might aid the passage of the probe include changing the neck position, realigning the TEE probe, and applying additional jaw thrust by elevating the angles of the mandible. The probe may also be passed with the assistance of laryngoscopy. The probe should never be forced past an obstruction. This could result in airway trauma or esophageal perforation.
Comprehensive Intraoperative Multiplane Transesophageal Echocardiographic Examination
Probe Manipulation: Descriptive Terms and Technique
The process of obtaining a comprehensive intraoperative multiplane TEE examination begins with a fundamental understanding of the terminology and technique for probe manipulation ( Fig. 11.1 ). Efficient probe manipulation minimizes esophageal injury and facilitates the process of acquiring and sweeping through 2D image planes. Horizontal imaging planes are obtained by moving the TEE probe up and down (proximal and distal) in the esophagus at various depths relative to the incisors ( upper esophageal [UE] : 20–25 cm; midesophageal [ME] : 30–40 cm; TG : 40–45 cm; deep TG : 45–50 cm) ( Table 11.1 ). Vertical planes are obtained by manually turning the probe to the patient’s left or right. Further alignment of the imaging plane can be obtained by manually rotating one of the two control wheels on the probe handle, which flexes the probe tip to the left or right direction or in the anterior or posterior plane. Multiplane probes may further facilitate interrogation of complex anatomic structures, such as the mitral valve (MV), by allowing up to 180 degrees of axial rotation of the imaging plane without manual probe manipulation.
View | Midesophageal Five-Chamber |
Multiplane angle range | 0–20 degrees |
Anatomy imaged | Left ventricular outflow tract Left ventricle and atrium Right ventricle and atrium Mitral and tricuspid valves Interatrial and interventricle septa |
Clinical utility | Ventricle function: global and regional Intracardiac chamber masses: thrombus, tumor, air; foreign bodies Mitral and tricuspid valve evaluation: pathologic and pathophysiologic conditions Congenital or acquired interatrial and ventral septal defects Hypertrophic obstructive cardiomyopathy evaluation Ventricular diastolic evaluation via transmitral and pulmonary vein Doppler-flow profile analysis Pericardial evaluation: pericarditis; pericardial effusion |
View | Midesophageal Four-Chamber |
Multiplane angle range | 0–20 degrees |
Anatomy imaged | Left ventricle and atrium Right ventricle and atrium Mitral and tricuspid valves Interatrial and interventricle septa Left pulmonary veins Right pulmonary veins Coronary sinus |
Clinical utility | Ventricle function: global and regional Intracardiac chamber masses: thrombus, tumor, air; foreign bodies Mitral and tricuspid valve evaluation: pathologic and pathophysiologic conditions Congenital or acquired interatrial and ventral septal defects Hypertrophic obstructive cardiomyopathy evaluation Ventricular diastolic evaluation via transmitral and pulmonary veins Doppler-flow profile analysis Pericardial evaluation: pericarditis; pericardial effusion Coronary sinus evaluation: coronary sinus catheter placement; dilation secondary to persistent left superior vena cava |
View | Midesophageal Mitral Commissural |
Multiplane angle range | 60–70 degrees |
Anatomy imaged | Left ventricle and atrium Mitral valve |
Clinical utility | Left ventricle function: global and regional Left ventricle and atrial masses: thrombus, tumor, air; foreign bodies Mitral valve evaluation: pathologic and pathophysiologic conditions Ventricular diastolic evaluation via transmitral Doppler-flow profile analysis |
View | Midesophageal Two-Chamber |
Multiplane angle range | 80–100 degrees |
Anatomy imaged | Left ventricle, atrium, atrial appendage Mitral valve Left pulmonary veins Coronary sinus |
Clinical utility | Left ventricle function: global and regional Left ventricle and atrial masses: thrombus, tumor, air; foreign bodies Mitral valve evaluation: pathologic and pathophysiologic conditions Ventricular diastolic evaluation via transmitral and pulmonary vein Doppler-flow profile analysis Coronary sinus evaluation: coronary sinus catheter placement; dilation secondary to persistent left superior vena cava |
View | Midesophageal Long Axis |
Multiplane angle range | 120–160 degrees |
Anatomy imaged | Left ventricle and atrium Left ventricular outflow tract Aortic valve Mitral valve Ascending aorta |
Clinical utility | Left ventricle function: global and regional Left ventricle and atrial masses: thrombus, tumor, air; foreign bodies Mitral valve evaluation: pathologic and pathophysiologic conditions Ventricular diastolic evaluation via transmitral Doppler-flow profile analysis Aortic valve evaluation: pathologic and pathophysiologic conditions Ascending aorta pathologic conditions: atherosclerosis, aneurysms, dissections Hypertrophic obstructive cardiomyopathy evaluation |
View | Midesophageal Aortic Valve Long Axis |
Multiplane angle range | 120–160 degrees |
Anatomy imaged | Aortic valve Proximal ascending aorta Left ventricular outflow tract Mitral valve Right pulmonary artery |
Clinical utility | Aortic valve: pathologic and pathophysiologic conditions Ascending aorta pathologic conditions: atherosclerosis, aneurysms, dissections Mitral valve evaluation: pathologic and pathophysiologic conditions |
View | Midesophageal Ascending Aorta Long Axis |
Multiplane angle range | 100–150 degrees |
Anatomy imaged | Ascending aorta Right pulmonary artery |
Clinical utility | Ascending aorta pathologic conditions: atherosclerosis, aneurysms, dissections Anterograde cardioplegia delivery evaluation Pulmonary embolus, thrombus |
View | Midesophageal Ascending Aortic Short Axis |
Multiplane angle range | 0–60 degrees |
Anatomy imaged | Ascending aorta Superior vena cava (short axis) Main pulmonary artery Right pulmonary artery Left pulmonary artery Pulmonic valve |
Clinical utility | Ascending aorta pathologic conditions: atherosclerosis, aneurysms, dissections Pulmonic valve: pathologic and pathophysiologic conditions Pulmonary embolus, thrombus evaluation Superior vena cava pathologic conditions: thrombus, sinus venosus atrial septal defect Pulmonary artery catheter placement |
View | Midesophageal Right Pulmonary Vein |
Multiplane angle range | 0–30 degrees |
Anatomy imaged | Midascending aorta Superior vena cava Right pulmonary vein |
Clinical utility | Ascending aorta dissection, aneurysm, plaque Superior vena cava thrombus Right pulmonary vein Doppler-flow velocity |
View | Midesophageal Aortic Valve Short Axis |
Multiplane angle range | 30–60 degrees |
Anatomy imaged | Aortic valve Interatrial septum Coronary ostia and arteries Right ventricular outflow tract Pulmonary valve |
Clinical utility | Aortic valve: pathologic and pathophysiologic conditions Ascending aorta pathologic conditions: atherosclerosis, aneurysms, dissections Left and right atrial masses: thrombus, embolus, air, tumor, foreign bodies Congenital or acquired interatrial septal defects evaluation |
View | Midesophageal Right Ventricular Inflow-Outflow (“Wraparound”) |
Multiplane angle range | 60–90 degrees |
Anatomy imaged | Right ventricle and atrium Left atrium Tricuspid valve Aortic valve Right ventricular outflow tract Pulmonic valve and main pulmonary artery |
Clinical utility | Right ventricle and atrial masses and left atrial: thrombus, embolus, tumor, foreign bodies Pulmonic valve and subpulmonic valve: pathologic and pathophysiologic conditions Pulmonary artery catheter placement Tricuspid valve: pathologic and pathophysiologic conditions Aortic valve: pathologic and pathophysiologic conditions |
View | Midesophageal Modified Bicaval Tricuspid Valve |
Multiplane angle range | 50–70 degrees |
Anatomy imaged | Right and left atria Superior vena cava (long axis) Inferior vena cava orifice Interatrial septum Right pulmonary veins Coronary sinus and Thebesian valve Eustachian valve Tricuspid valve |
Clinical utility | Right and left atrial masses: thrombus, embolus, air, tumor, foreign bodies Superior vena cava pathologic conditions: thrombus, sinus venosus atrial septal defect Inferior vena cava pathologic conditions: thrombus, tumor Femoral venous line placement Coronary sinus catheter line placement Right pulmonary vein evaluation: anomalous return, Doppler evaluation for left ventricular diastolic function Congenital or acquired interatrial septal defects evaluation Pericardial effusion evaluation Tricuspid valve evaluation for stenosis, regurgitation, and calculated estimation of pulmonary artery pressures from regurgitant Doppler-flow velocity profile |
View | Midesophageal Bicaval |
Multiplane angle range | 80–110 degrees |
Anatomy imaged | Right and left atria Superior vena cava (long axis) Inferior vena cava orifice: advance probe and turn to right to visualize inferior vena cava in the long axis, liver, hepatic and portal veins Interatrial septum Right pulmonary veins: turn probe to right Coronary sinus and Thebesian valve Eustachian valve |
Clinical utility | Right and left atrial masses: thrombus, embolus, air, tumor, foreign bodies Superior vena cava pathologic conditions: thrombus, sinus venosus atrial septal defect Inferior vena cava pathologic conditions: thrombus, tumor Femoral venous line placement Coronary sinus catheter line placement Right pulmonary vein evaluation: anomalous return, Doppler evaluation for left ventricular diastolic function Congenital or acquired interatrial septal defects evaluation Pericardial effusion evaluation |
View | Upper Esophageal Right and Left Pulmonary Veins |
Multiplane angle range | 90–100 degrees |
Anatomy imaged | Pulmonary veins Pulmonary artery Ascending aorta |
Clinical utility | Pulmonary vein pathologic condition Ascending aortic aneurysm, dissection Pulmonary embolism, thrombus |
View | Midesophageal Left Atrial Appendage |
Multiplane angle range | 90–110 degrees |
Anatomy imaged | Left pulmonary veins Left atrial appendage |
Clinical utility | Left pulmonary vein Doppler-flow velocity Left atrial appendage thrombus |
View | Midesophageal Ascending Aortic Short Axis |
Multiplane angle range | 0–60 degrees |
Anatomy imaged | Ascending aorta Superior vena cava (short axis) Main pulmonary artery Right pulmonary artery Left pulmonary artery Pulmonic valve |
Clinical utility | Ascending aorta pathologic conditions: atherosclerosis, aneurysms, dissections |
View | Transgastric Basal Short Axis |
Multiplane angle range | 0–20 degrees |
Anatomy imaged | Left and right ventricles Mitral valve Tricuspid valve |
Clinical utility | Mitral valve evaluation (“fish-mouth view”): pathologic, pathophysiologic conditions Tricuspid valve evaluation: pathologic, pathophysiologic conditions Basal left ventricular regional function Basal right ventricular regional function |
View | Transgastric Midpapillary |
Multiplane angle range | 0–20 degrees |
Anatomy imaged | Left and right ventricles Papillary muscles |
Clinical utility | Mid left and right ventricular regional and global functions Intracardiac volume status |
View | Transgastric Apical Short Axis |
Multiplane angle range | 0–20 degrees |
Anatomy imaged | Left and right ventricles |
Clinical utility | Apical left and right ventricular regional functions Ventricular aneurysm |
View | Transgastric Right Ventricular Basal |
Multiplane angle range | 0–20 degrees |
Anatomy imaged | Left and right ventricle Right ventricular outflow tract Tricuspid valve (short axis) Pulmonic valve |
Clinical utility | Left and right ventricular regional and global functions Intracardiac volume status Tricuspid valve pathologic condition Pulmonic valve regurgitation and stenosis evaluation |
View | Transgastric Right Ventricular Inflow-Outflow |
Multiplane angle range | 60–90 degrees |
Anatomy imaged | Right ventricle and right atrium Left atrium Tricuspid valve Aortic valve Right ventricular outflow tract Pulmonic valve and main pulmonary artery |
Clinical utility | Right ventricle and atrial masses and left atrial: thrombus, embolus, tumor, foreign bodies Pulmonic valve and subpulmonic valve: pathologic, pathophysiologic conditions Pulmonary artery catheter placement Tricuspid valve: pathologic, pathophysiologic conditions Aortic valve: pathologic, pathophysiologic conditions |
View | Transgastric Two-Chamber |
Multiplane angle range | 80–100 degrees |
Anatomy imaged | Left ventricle and left atrium Mitral valve: chordae and papillary muscles Coronary sinus |
Clinical utility | Left ventricular regional and global functions (including apex) Left ventricular and atrial masses: thrombus, embolus, air, tumor, foreign bodies Mitral valve: pathologic, pathophysiologic conditions |
View | Transgastric Right Ventricular Inflow |
Multiplane angle range | 100–120 degrees |
Anatomy imaged | Right ventricle and right atrium Tricuspid valve: chordae and papillary muscles |
Clinical utility | Right ventricular regional and global functions Right ventricular and atrial masses: thrombus, embolus, tumor, foreign bodies Tricuspid valve: pathologic, pathophysiologic conditions |
View | Transgastric Long Axis |
Multiplane angle range | 90–120 degrees |
Anatomy imaged | Left ventricle and outflow tract Aortic valve Mitral valve |
Clinical utility | Left ventricular regional and global functions Mitral valve: pathologic, pathophysiologic conditions Aortic valve: pathologic, pathophysiologic conditions |
View | Deep Transgastric Long Axis |
Multiplane angle range | 0–20 degrees (anteflexion) |
Anatomy imaged | Left ventricle and outflow tract Interventricular septum Aortic valve and ascending aorta Left atrium Mitral valve Right ventricle Pulmonic valve |
Clinical utility | Aortic and subaortic valve: pathologic, pathophysiologic conditions Mitral valve: pathologic, pathophysiologic conditions Left and right ventricular global functions Left and right ventricular masses: thrombus, embolus, tumor, foreign bodies Congenital or acquired interventricular septal defect evaluation |
View | Upper Esophageal Aortic Arch: Long Axis |
Multiplane angle range | 0 degrees |
Anatomy imaged | Aortic arch; left brachiocephalic vein; left subclavian and carotid arteries; right brachiocephalic artery |
Clinical utility | Ascending aorta and arch pathologic conditions: atherosclerosis, aneurysms, dissections; aortic cannulation site evaluation for cardiopulmonary bypass |
View | Upper Esophageal Aortic Arch: Short Axis |
Multiplane angle range | 90 degrees |
Structures imaged | Aortic arch; left brachiocephalic vein; left subclavian and carotid arteries; right brachiocephalic artery Main pulmonary artery and pulmonic valve |
Clinical utility | Ascending aorta and arch pathologic conditions: atherosclerosis, aneurysms, dissections Pulmonary embolus; pulmonary valve evaluation (insufficiency, stenosis, Ross procedure); pulmonary artery catheter placement |
View | Descending Aorta Short Axis |
Multiplane angle range | 0 degrees |
Anatomy imaged | Descending thoracic aorta Left pleural space |
Clinical utility | Descending aorta pathologic conditions: atherosclerosis, aneurysms, dissections Intraaortic balloon placement evaluation Left pleural effusion |
View | Descending Aorta Long Axis |
Multiplane angle range | 90–110 degrees |
Anatomy imaged | Descending thoracic aorta Left pleural space |
Clinical utility | Descending aorta pathologic conditions: atherosclerosis, aneurysms, dissections Intraaortic balloon placement evaluation Left pleural effusion |
Comprehensive Intraoperative Transesophageal Echocardiographic Examination: Imaging Planes and Structural Analysis
Left and Right Ventricles
The left ventricle should be examined carefully for global and regional function using multiple transducer planes, depths, and rotational and angular orientations ( Fig. 11.2 ). Analysis of segmental function is based on a qualitative visual assessment that includes the following grading system of both LV wall thickness and motion (endocardial border excursion) during systole: 1 = normal (>30% thickening); 2 = mild hypokinesis (between 10% and 30% thickening); 3 = severe hypokinesis (<10% thickening); 4 = akinesis (no thickening); 5 = dyskinesis (paradoxic motion). The recently recommended ME five-chamber view enables visualization of the septal and lateral walls (slightly anterior) of the left ventricle at 0 to 20 degrees from its base to the apex, along with the left ventricular outflow tract (LVOT), right ventricle and both atria (see Fig. 11.2 ). Slight TEE probe advancement eliminates the LVOT from the image window and permits the development of the ME four-chamber view (see Fig. 11.2 ), demonstrating a slightly more mid-to-inferior plane. TEE probe rotation to approximately 80 to 100 degrees develops the ME two-chamber view (see Fig. 11.2 ), which removes the right-sided chambers from the imaging window but enables visualization of the inferior and anterior LV walls at the basal, mid, and apical levels segments. The ME long-axis (LAX) view at 120 to 160 degrees (see Fig. 11.2 ) allows evaluation of the remaining anteroseptal and inferolateral (posterior) LV segments. Because the left ventricle is usually oriented inferiorly to the true horizontal plane, slight retroflexion of the probe tip may be required to minimize LV foreshortening. The TG midpapillary SAX view (TG mid-SAX) at 0 to 20 degrees (see Fig. 11.2 ) is the most commonly used view for monitoring LV function, because it allows a midpapillary assessment of the LV segments supplied by the corresponding coronary arteries (right coronary artery, left circumflex, and left anterior descending [LAD]). This view also enables qualitative and quantitative evaluation of pericardial effusions. Advancing or withdrawing the probe at the TG depth enables LV evaluation at the respective newly recommended TG apical SAX and basal levels ( TG basal SAX ) (see Fig. 11.2 ), respectively. Further evaluation of the left ventricle can be obtained at the midpapillary TG depth by rotating the probe forward to the TG two-chamber view (80 to 100 degrees) ( Fig. 11.2 ) and TG LAX (90 to 120 degrees) (see Fig. 11.2 ).
Right ventricular (RV) regional and global function can be assessed from the ME five-chamber and four-chamber views (see Fig. 11.2 ), which allows visualization of the septal and free walls. Although a formal segmental scheme has not been developed for the RV free wall, regional assessment of the septum can be performed. Turning the probe to the right and advancing slightly from the ME depth allows visualization of the tricuspid valve (TV), coronary sinus (CS), and RV apex. Rotating the probe between 60 and 90 degrees reveals the ME RV inflow-outflow view (see Fig. 11.2 ), in which the right atrium (RA), TV, inferior RV free wall, right ventricular outflow tract (RVOT), pulmonic valve (PV), and main pulmonary artery (PA) can be viewed wrapping around the centrally oriented aortic valve (AV). This view often allows optimal Doppler beam alignment to evaluate the TV and can also be helpful for directing PA catheter floating and positioning. The same right-sided structures can also be visualized from a different perspective by advancing the probe to the TG depth to obtain the newly recommended TG RV inflow-outflow view (see Fig. 11.2 ). The TG midpapillary SAX view (see Fig. 11.2 ) displays the crescent-shaped, thinner-walled right ventricle to the left of the left ventricle. Slightly withdrawing the probe reveals the right ventricle at a more basal level along with the PV in the newly recommended TG RV basal view (see Fig. 11.2 ). The TG RV inflow view (see Fig. 11.2 ) is developed by turning the probe to the right to center the right ventricle at this depth and rotating the multiplane angle forward to 100 to 120 degrees, thereby revealing the inferior RV free wall.
Mitral Valve
The echocardiographic evaluation of the MV requires a thorough assessment of its leaflets (anterior and posterior), annulus, and the subvalvular apparatus (chordae tendineae, papillary muscles, and adjacent LV walls) to locate lesions and to define the cause and severity of the pathophysiologic condition. The mitral leaflets can be further divided into posterior leaflet scallops: lateral (P1), middle (P2), and medial (P3), which correspond with respective anterior leaflet sections: lateral third (A1), middle third (A2), and medial third (A3). The leaflets are united at the anterolateral and posteromedial commissures. The ME four-chamber view (see Fig. 11.2 ) displays the larger appearing anterior leaflet (A2,3) to the left of the posterior leaflet (P2,1), whereas the ME five-chamber view reveals more of A1 and P1. Anteflexing the probe provides imaging of the anterolateral aspect of the MV, whereas gradual advancement of the probe and retroflexion shift the image plane to the posteromedial aspect of MV. Maintaining the probe at the ME depth and rotating the multiplane angle forward to 60 to 70 degrees develops the ME mitral commissural view (see Fig. 11.2 ), in which A2 is flanked by P1 on the right and P3 on the left, giving A2 the appearance of a “trap-door” as it moves in and out of the imaging plane throughout the cardiac cycle. Farther forward rotation of the probe to 80 to 100 degrees develops the ME two-chamber view (see Fig. 11.2 ), revealing P3 to the left and A1 on the right. Final forward probe rotation to 120 to 160 degrees reveals the ME LAX view (see Fig. 11.2 ), which images P2 on the left and A2 on the right. The TG basal SAX view (see Fig. 11.2 ) enables visualization of both MV leaflets (“fish-mouth view”) if the probe is anteflexed and withdrawn slightly from the midpapillary level of the left ventricle. In this view, the posteromedial commissure is in the upper left, the anterolateral commissure is in the lower right, the posterior leaflet is to the right, and the anterior leaflet is to the left of the displayed image. Rotation of the probe to 80 to 100 degrees develops the TG two-chamber view (see Fig. 11.2 ) that is especially useful for evaluating the chordae tendineae and corresponding papillary muscles.
Aortic Valve, Aortic Root, and Left Ventricular Outflow
The three cusps of the semilunar AV are best visualized simultaneously in the ME AV SAX view (see Fig. 11.2 ), which is obtained by rotating the probe forward to 30 to 60 degrees. The noncoronary cusp is superior, lying adjacent to the atrial septum; the right cusp is inferior; and the left cusp lies to the right, pointing in the direction of the left atrial appendage (LAA). This view permits planimetry of the AV orifice, evaluation of congenital anomalies of the AV (eg, bicuspid AV), and qualitative assessment of aortic insufficiency (AI) when CFD is used. The ME AV LAX view (see Fig. 11.2 ) can be obtained at the same depth while rotating the probe to 120 to 160 degrees, allowing for visualization of the LVOT, AV annulus, and leaflets (right and either noncoronary or left), sinuses of Valsalva, sinotubular junction, and proximal ascending aorta. This view is particularly useful for evaluating AI with CFD, systolic anterior motion of the MV, and proximal aortic pathologic conditions (eg, dissections, aneurysms). Rotating the probe back to 90 to 120 degrees and advancing into the stomach to TG level develops the TG LAX view (see Fig. 11.2 ). In this view, the LVOT and AV are oriented to the right and inferiorly in the displayed image, thereby providing an optimal window for parallel Doppler beam alignment for the assessment of flows and pressure gradients (aortic stenosis [AS], hypertrophic obstructive cardiomyopathy). Rotating the probe back farther to 0 to 20 degrees, advancing deep into the stomach, and anteflexing the tip so that it lies adjacent to the LV apex, allows for the development of the deep TG LAX view, now referred to as the deep TG five-chamber view (see Fig. 11.2 ), which also provides optimal Doppler beam alignment for measuring transaortic valve and LVOT flow velocities and may provide an additional window for assessing flows through muscular ventricular septal defects and LV apical pathologic conditions (thrombus, aneurysms).
Tricuspid Valve
The echocardiographic evaluation of the TV requires a thorough assessment of its three leaflets (anterior, posterior, and septal), annulus, chordae tendineae, papillary muscles, and the corresponding RV walls. In the ME five-chamber view, the septal TV leaflet is displayed on the right side, and the anterior leaflet is usually on the left side of the annulus. Advancing the probe slightly reveals the ME four-chamber view (see Fig. 11.2 ), with the septal TV leaflet on the right side and the posterior TV leaflet usually on the left side of the annulus. Rotating the multiplane angle to 50 to 70 degrees develops the ME RV inflow-outflow view (see Fig. 11.2 ), which displays the posterior TV leaflet on the left side of the image and the anterior TV leaflet on the right side of the image adjacent to the AV. Slightly turning the probe rightward from the ME bicaval view permits the development of the newly recommended ME-modified bicaval TV view (see Fig. 11.2 ) with the anterior leaflet on the right and the posterior leaflet on the left. The ME-modified bicaval view often provides better alignment of a CWD beam with a tricuspid regurgitation (TR) jet for estimating PA pressures. The TG RV inflow view (see Fig. 11.2 ) is obtained by advancing the probe into the stomach and rotating to 100 to 120 degrees. This view is ideal for visualizing the chordae tendineae and papillary muscles in the right ventricle. Rotating back to the TG mid-SAX at 0 to 20 degrees and slightly withdrawing the probe to obtain the TG RV basal view provides a cross-sectional, SAX view of the TV, displaying the anterior leaflet in the far field, the posterior leaflet to the left in the near field, and the septal leaflet on the right side of the image.
Pulmonic Valve and Pulmonary Artery
The PV is a trileaflet, semilunar valve. The ME AV SAX view (see Fig. 11.2 ) displays the transition between the RVOT and PV. Rotating the probe back toward 0 degrees and withdrawing slightly develops the ME ascending aortic SAX view (see Fig. 11.2 ) displaying the transition between the PV and the main PA and its bifurcation. Although the right PA is usually easy to visualize by turning the probe to the right, the left PA is often obscured by the interposing, air-filled, left mainstem bronchus. The ME RV inflow-outflow view (see Fig. 11.2 ) can also be used to assess the PV and main PA, which lie on the right side of the image adjacent to the AV. However, the newly recommended TG RV inflow-outflow view (see Fig. 11.2 ), with the PV on the right side of the screen and the more reliable UE aortic arch SAX view (see Fig. 11.2 ), displays the PV oriented to the left of the cross-sectional view of the aortic arch and usually provides a more parallel Doppler beam orientation through for the evaluation of pulmonic regurgitation or stenosis.
Left Atrium, Left Atrial Appendage, Pulmonary Veins, and Atrial Septum
The left atrium is the closest cardiac structure to the TEE probe when positioned in the esophagus. Consequently, the left atrium is usually easily displayed in the superior aspect of the 2D image sector. The ME five-chamber and four-chamber views (see Fig. 11.2 ) display the left atrium almost in its entirety with the LAA oriented to its superior and lateral aspects when the probe is slightly withdrawn. The muscular ridges of the pectinate muscles within the LAA should not be confused with thrombi. A slightly farther withdrawal of the probe, turning it to the left, and rotating the array to approximately 90 degrees, develops the newly defined UE left pulmonary vein view (see Fig. 11.2 ), which allows the left upper pulmonary vein (LUPV) to be imaged as it enters the left atrium from the anterior-to-posterior direction and separated from the lateral border of the LAA by the warfarin ridge . Turning the probe to the right at this depth reveals the newly defined UE right pulmonary view (see Fig. 11.2 ), and a slight advancement and rotation of the array to 0 degrees permits visualization of the newly defined ME right pulmonary vein view (see Fig. 11.2 ), which both reveal the right upper pulmonary vein (RUPV) entering the left atrium in an anterior-to-posterior direction and the right PA or superior vena cava (SVC), respectively.
The interatrial septum (IAS), consisting of thicker limbus regions flanking the thin fossa ovalis, can also be imaged in the ME four-chamber view (see Fig. 11.2 ). Benign lipomatous hypertrophy of the IAS must be distinguished from pathologic lesions such as atrial myxomas. The patency of the IAS and the presence of a patent foramen ovale (PFO) or congenital atrial septal defects should be assessed with Doppler echocardiography and intravenous injections of agitated saline. Advancing and rotating the probe to 80 to 100 degrees develops the ME two-chamber view (see Fig. 11.2 ), which allows for further imaging of the left atrium from left to right. The LAA and LUPV can be seen by turning the probe slightly to the left to develop the newly defined ME LAA view (see Fig. 11.2 ). Rotating the probe to the right at this level and adjusting the multiplane angle to 80 to 110 degrees will develop the ME bicaval view (see Fig. 11.2 ), which delineates the SVC entering the RA to the right of the image and the inferior vena cava (IVC) entering from the left. The IAS can be seen in the middle of the image separating the left and right atria.
Right Atrium and Coronary Sinus
The RA can be most easily visualized in the ME five-chamber and ME four-chamber views (see Fig. 11.2 ) by turning the probe to the patient’s right side, as well as in the ME RV inflow-outflow view (see Fig. 11.2 ). In these views, the entire RA can be visualized for size, overall function, and the presence of masses (thrombi, tumors). Rotating the multiplane angle to 80 to 110 degrees develops the ME bicaval view (see Fig. 11.2 ), which displays the RA and its internal structures (Eustachian valve, Chiari network, crista terminalis). The SVC can be imaged entering the RA on the right, superior to the right atrial appendage, and the IVC enters the RA on the left of the display. Advancing and turning the probe to the right will allow for a qualitative evaluation of the intrahepatic segment of the IVC and hepatic veins. At the TG depth, both the TG RV inflow-outflow and TG RV inflow views may provide more optimal imaging windows for visualizing the RA and collateral structures. Pacemaker electrodes and central venous catheters for hemodynamic monitoring or cardiopulmonary bypass (CPB) can be easily imaged in this view.
The CS lies posteriorly in the atrioventricular groove, emptying into the RA at the inferior extent of the atrial septum. The CS can be viewed in LAX entering the RA just superior to the tricuspid annulus by advancing and slightly retroflexing the probe from ME four-chamber view (see Fig. 11.2 ). The CS can be imaged cross-sectionally in the SAX in the ME two-chamber view (see Fig. 11.2 ) in the upper left of the display. The CS and Thebesian valve can also be visualized in the ME-modified bicaval view (see Fig. 11.2 ) on the upper left of the image as it enters the RA at an obtuse angle. Echocardiographic visualization of the CS can be useful for directing the placement of CS catheters used for CPB.
Thoracic Aorta
The proximal and midascending thoracic aorta can be visualized in the SAX in the ME ascending aortic SAX view (see Fig. 11.2 ). Advancing and withdrawing the probe should enable visualization of the thoracic aorta from the sinotubular junction to a point 4- to 6-cm superior to the AV and allow an inspection for aneurysms and dissections. Rotating the multiplane angle to 100 to 150 degrees develops the ME ascending aortic LAX view (see Fig. 11.2 ), which optimally displays the parallel anterior and posterior walls for measuring proximal and midascending aortic diameters. This display can also be obtained from the ME AV LAX view (see Fig. 11.2 ) by slightly withdrawing and turning the probe to the left.
TEE imaging of the aortic arch is often obscured by the interposing, air-filled trachea. The most optimal views of the aortic arch are obtained by withdrawing the probe from the ME ascending aortic SAX view at 0 degrees and rotating to the left to obtain the UE aortic arch LAX view (see Fig. 11.2 ), which displays the proximal arch followed by the mid-arch, the great vessels (brachiocephalic, left carotid artery, and left subclavian artery), and the distal arch before it joins the proximal descending thoracic aorta imaged in cross-section. Alternatively, rotating the probe to 90 degrees develops the UE aortic arch SAX view (see Fig. 11.2 ). Turning the probe to the left in this view delineates the transition of the distal arch with the proximal descending thoracic aorta. Turning the probe to the right and slightly withdrawing it will allow for the mid-arch and great vessels to be imaged on the right side of the screen, followed by the distal ascending aorta when the probe is subsequently advanced and rotated forward to the 120-degree ME ascending aortic LAX view (see Fig. 11.2 ). Epiaortic aortic scanning may be particularly useful for assessing the extent of ascending aortic and arch pathologic conditions (ie, aneurysms, dissection, atherosclerosis) to determine cross-clamping and cannulation sites for CPB.
A SAX image of the descending thoracic aorta is obtained by turning the probe leftward from the ME four-chamber view to produce the descending aortic SAX view (see Fig. 11.2 ). Rotating the multiplane angle of the probe from 0 to 90 to 110 degrees produces an LAX image, the descending aortic LAX view (see Fig. 11.2 ). The descending thoracic aorta should be interrogated in its entirety, beginning at the distal aortic arch, by continually advancing the probe and turning slightly to the left until the celiac and superior mesenteric arteries are visualized branching tangentially from the anterior surface of abdominal aorta when the probe is in the stomach. A thorough examination of the descending thoracic aorta may be necessary to evaluate the distal extent of an aneurysm or dissection. In addition, the descending aortic SAX and LAX views can be useful for confirming appropriate intraaortic balloon positioning.
Clinical Applications
Left Ventricular Assessment
Assessment of Left Ventricular Size
LV volume measurements may be with biplane disk summary or area-length measurements using 2D echocardiography or using 3D data sets. Since the biplane disk summation method (modified Simpson method) corrects for shape distortions, it is currently the recommended method of 2D volume measurements. With biplane disk summation, the total LV volume is calculated from the summation of a stack of elliptical disks. The height of each disk is calculated as a fraction of the LV LAX, based on the longer of the two lengths from the two- and four-chamber views. The cross-sectional area (CSA) of the disk is based on the diameters obtained, and the volume of the disk is estimated. The volume is obtained by the summation of these values.
Left Ventricular Preload by End-Diastolic Dimensions
Preload is often estimated by measuring left-sided heart filling pressures (pulmonary capillary wedge pressure [PCWP], left atrial pressure [LAP], or left ventricular end-diastolic pressure [LVEDP]) in conventional hemodynamics, measuring LV end-diastolic dimensions. It has been proposed that end-diastolic dimensions provide a better index of preload than the PCWP. When PCWP and end-diastolic volume (EDV), derived from SAX areas at the level of the papillary muscles, were compared as predictors of cardiac index (CI) in patients undergoing coronary artery bypass grafting (CABG) surgery, a strong correlation was observed between end-diastolic area (EDA) or EDV and CI, whereas no significant correlation was found between PCWP and CI.
TEE is often, for practical reasons, limited to a single SAX view at the level of the papillary muscles. Some evidence suggests that SAX EDAs measured at this level correlate reasonably well with measurements obtained by on-heart echocardiography and with EDVs measured simultaneously using radionuclides. There are two main echocardiographic signs of decreased preload:
- 1.
Decrease in EDA (<5.5 cm 2 /m 2 ) invariably reflects hypovolemia.
- 2.
Obliteration of the end-systolic area (the “kissing ventricle sign”) that accompanies the decrease in EDA in severe hypovolemia.
Left Ventricular Systolic Function
The echocardiographic assessment of global and regional LV function consists of 2D, 3D, or Doppler evaluation of cardiac structures. Using echocardiography, contractility has most frequently been estimated utilizing end-diastolic and end-systolic dimensions.
With 2D echocardiography, multiple tomographic cuts can be obtained and used to calculate ventricular volumes using a variety of formulas such as the modified Simpson formula. Using the ventricular volumes, ejection fraction (EF) can be calculated using the standard formula:
where LVEDV is LV end-diastolic volume, and LVESV is LV end-systolic volume.
During intraoperative TEE, it is most convenient to monitor a single, SAX view at the level of the midpapillary muscles. Once the end-diastolic and end-systolic endocardial areas have been delineated with the help of tracing software, contractility may be estimated using the fractional area of contraction (FAC) or the ejection fraction area (EFA):
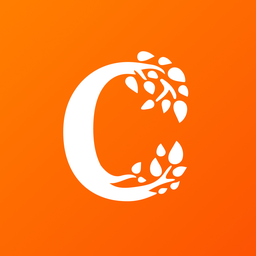
Full access? Get Clinical Tree
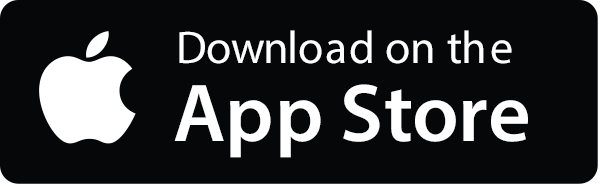
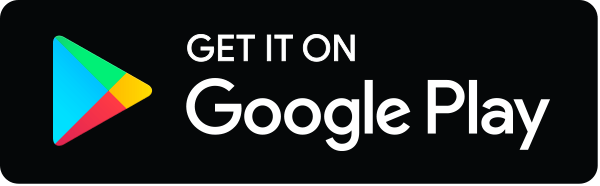