Barotrauma and Bronchopleural Fistula: Introduction
This chapter considers the possible origins of extraalveolar air in ventilated patients and then reviews the manifestations and most frequent clinical settings of its various forms. After a discussion of general principles of management and a review of reported therapeutic approaches, it presents logical steps for prevention in susceptible patients and points out important gaps in the existing evidence base pertaining to this important topic. Although all the clinical forms of barotrauma are touched upon, most attention is devoted to those that pose a threat to life.
Only overt extraalveolar air is covered in this chapter. The reader is referred to Chapter 42 for a discussion of lung damage at the tissue or subcellular level related to mechanical lung distension and the application of positive pressure to the airways. Because data from laboratory studies are covered extensively in that discussion, this chapter deals primarily with barotrauma as a complication in patients, referring mainly to the adult clinical literature. As will be apparent, although the latter is replete with anecdotes and observational reports, this focus primarily on human data means that the evidence base in terms of prospective studies and “hard data” available to the clinician is remarkably limited. Spontaneous pneumothorax and other forms of extraalveolar air encountered in patients who are not intubated or receiving mechanical ventilation are not dealt with extensively here, nor is decompression-related barotrauma or bronchopleural fistula complicating lung resection.
Definitions
Pneumothorax, subcutaneous emphysema, and other clinical forms of extraalveolar air occurring in association with mechanical ventilation are commonly referred to as barotrauma. This term is doubly unfortunate, trauma connoting iatrogenic injury and baro implying that it is pressure, rather than volume, shear force, or some other factor that produces it. In fact, these implications of both roots of the word are probably incorrect, and the expression ventilator-associated extraalveolar air would be technically more appropriate. Similarly, the term bronchopleural air leak would be more accurate than bronchopleural fistula, because of the implications of inflammation and suppuration associated with the word fistula in surgical and other settings. Like barotrauma, however, the latter is so ingrained in clinical usage that change is unlikely, and the more familiar terms are used in this chapter, as elsewhere in this book.
Although extraalveolar air appearing during ventilator support may not be caused by the ventilator itself, the expression ventilator-induced lung injury would seem as applicable to clinical barotrauma as to parenchymal damage associated with mechanical stretch and overdistension. Again, however, owing more to convention than to logical etymology, the term ventilator-induced lung injury is generally reserved for the latter, in this book and in the broader literature.
Usually, barotrauma in mechanically ventilated patients is automatically assumed to be a complication of mechanical ventilation. As with nosocomial pneumonia, however, ventilatory muscle dysfunction, and most of the other adverse developments to which ventilated patients are prone, whether the ventilator per se is responsible is usually unclear, because patients ill enough to require endotracheal intubation, supplemental oxygen, positive-pressure ventilation, and management in an intensive care unit (ICU) tend to have numerous other predispositions to such complications. Each of these things would be better thought of as complications associated with, rather than of, mechanical ventilation.1,2
Pathophysiology
Barotrauma in a patient receiving positive-pressure ventilation has a number of potential causes (Table 44-1).3 Most often extraalveolar air during mechanical ventilation results from overdistension of alveoli and rupture of their walls down a pressure gradient from airspace into bronchovascular sheath, as illustrated in Figure 44-1.4 This mechanism was worked out many years ago in elegant animal experiments by C.C. and M.T. Macklin:5,6 “… pulmonic interstitial emphysema and its sequelae—air in the mediastinum, peritoneal cavity, subcutaneous tissues, and pleural cavity—are present in many conditions; differing widely in their cause, their clinical manifestations and their seriousness. All of these conditions, however, have a single common factor, a gradient of pressure between the alveoli and vessel sheath, and hence an opportunity for air to gain access to the interstitial tissues of the lung.”6
|
Figure 44-1
Mechanism of alveolar rupture during mechanical ventilation. Pressures between adjacent alveoli equalize rapidly, but, especially in the presence of high alveolar volume, increased alveolar pressure, in comparison with that in the adjacent bronchovascular sheath, establishes a pressure gradient that may result in rupture of the alveolar wall, allowing passage of air into the interstitial tissue of the bronchovascular sheath. (Used, with permission, from Maunder et al.4)
According to the work of the Macklins,5,6 the basic requirement for alveolar rupture is the presence of a pressure gradient between the alveoli and their surrounding structures. Interalveolar walls are probably not susceptible to such pressure gradients, as they are very thin and the pressures between adjacent alveoli are probably equal. Any sudden increase in alveolar pressure (or presumably a fall in perivascular interstitial pressure), however, may establish a gradient sufficiently large to disrupt alveolar walls at their bases (see Fig. 44-1), introducing air into the pulmonary interstitium. Alveolar overdistension would tend to thin and stretch the alveolar membranes, facilitating rupture once the pressure gradient was established. This mechanism can readily be understood in the case of extraalveolar air following sudden deceleration injury, such as falling into water from a height,7 but it likely is the main means of alveolar rupture in the majority of other clinical settings as well.3,4,8
Spread of extraalveolar air via bronchovascular sheaths after alveolar rupture was dramatically demonstrated by Jamadar et al when pneumomediastinum developed in a patient undergoing liquid ventilation.9 After the liquid medium was removed from the bronchial tree and alveoli, computed tomography of the chest demonstrated persistence of the radiopaque perfluorocarbon fluid in the bronchovascular sheaths, confirming the mechanism elucidated a half-century earlier by the Macklins.
Beyond clinical barotrauma that occurs as a direct complication of mechanical ventilation, it is important to be cognizant of other possible sources not related to the ventilator itself (Table 44-2).3,8 Distinguishing these causes from barotrauma secondary to mechanical ventilation itself may be challenging in some circumstances, particularly when adequate clinical history is lacking. Pneumothorax appearing within the first few hours following initiation of mechanical ventilation may be the result of previous trauma, overinflation during manual ventilation, or procedures such as central line attempts before intubation. Even in patients already receiving ventilator support, the appearance of extraalveolar air may represent “pseudobarotrauma”—external trauma to the lung from attempts at placing central venous access or various endoscopic procedures—rather than “spontaneous” alveolar rupture.
|
Air originating in the upper respiratory tract can dissect downward from the head and neck and produce subcutaneous emphysema, pneumomediastinum, and conceivably even pneumothorax. During mechanical ventilation, this could occur in the presence of negative intrathoracic pressure, as with vigorous efforts against a partially occluded airway or in severe patient–ventilator dyssynchrony. Reported causes of pneumomediastinum from air dissection from above include facial or mandibular fractures,10 retropharyngeal abscess,11 and dental extractions, particularly if these involve the lower molar teeth and air-turbine drilling.12
Mediastinal air and other barotrauma can also originate in the intrathoracic airways, as after blunt or penetrating chest trauma,13,14 as a complication of bronchoscopic procedures, during percutaneous dilatational tracheotomy,15 or from perforation by a foreign body. Injury to the upper airway or laceration of the posterior membranous trachea may occur during attempted endotracheal intubation, particularly when a stylet is used, and this has been associated with clinical barotrauma in numerous reports.16–20
Endoscopic procedures21–23 and other interventions may rupture or perforate the esophagus, providing access for air into the mediastinum. In many instances, but not always, signs of mediastinitis accompany the appearance of pneumomediastinum following esophageal procedures.8 Air in the mediastinum is also a characteristic feature of Boerhaave syndrome,24 and the typical history of vigorous retching after a large meal may be absent.
Most often the origin of clinical barotrauma is the lung parenchyma, but even then the mechanism may be other than “spontaneous” alveolar rupture. Other possibilities include penetrating trauma that lacerates pulmonary tissue, surgical procedures involving the intrathoracic structures, bronchoscopic procedures, and transthoracic needle aspiration. When acute mediastinitis or pleural empyema involves gas-producing organisms, palpable or radiographically detectable extraalveolar air may result; gas arising from soft-tissue infections such as clostridial gangrene may also be confused with barotrauma.
Finally, exogenous air may find its way into the pleural space, subcutaneous tissues, or mediastinum. Most commonly this occurs during or following thoracentesis, chest tube insertion, tracheotomy,25 or mediastinoscopy. Rarely, air may enter the soft tissues following cutaneous injury to an extremity26 or elsewhere in the body, producing subcutaneous emphysema that could be confused with barotrauma.
For many years, a primary goal of mechanical ventilation was to limit peak airway pressures, based on the belief that high peak airway pressures were a primary mediator of barotrauma.27–31 The desire to avoid high peak airway pressures was a main driver in the introduction of pressure-targeted modes of ventilation and the different forms of high-frequency ventilation.3 As discussed in Chapter 42, however, a large body of evidence now supports the conclusion that it is excessive volume, not airway pressure per se, and especially not peak airway pressure measured outside the patient, that primarily determines the occurrence and severity of ventilator-induced lung injury.
Peak airway pressure during mechanical ventilation can be influenced by a number of factors (Table 44-3), most of which do not affect (or reflect) alveolar volume. This can readily be understood by envisioning the various potential sources for high peak airway pressure as sensed at the ventilator’s manometer. Increased resistance to airflow in the ventilator circuit or endotracheal tube, or in the patient’s conducting airways, will be reflected in an increased peak inspiratory airway pressure. Likewise, an increase in chest wall pressure, as with coughing, chest strapping, or massive generalized edema, will increase peak airway pressure without increasing transalveolar pressure or alveolar volume. Even increased pressure at the alveolar level should not cause disruption of alveolar walls unless this pressure is unevenly distributed between adjacent alveoli or between alveoli and the adjoining bronchovascular sheath.
|
This conclusion becomes obvious when one considers the common activities that markedly raise peak airway pressure without causing alveolar rupture.3 Normal persons routinely generate peak airway pressures up to 200 cm H2O or more during a cough or sneeze.32 In a study of fifty-six normal male subjects ranging in age from 6 to 64 years, Cook et al33 found their mean individual maximum peak expiratory airway pressures to be 237 ± 45 cm H2O. In that study, the subjects sustained these pressures for at least 1 to 2 seconds, and even longer periods of sustained high peak airway pressures can be maintained during the Valsalva maneuver, during which pressures as high as 200 torr (286 cm H2O) have been documented.34 Airway pressures well above 100 cm H2O are routinely achieved and held by trumpet players35 without causing barotrauma. Alveolar rupture is extremely rare in wind instrument players, as it is in glass blowers, weight lifters, and other healthy people after coughing, sneezing, or straining.3,36
In addition, for any given airway resistance, varying inspiratory flow will also vary the pressure developed as a given volume is delivered to a patient’s lungs, but will not affect end-inspiratory alveolar pressure. For any given volume-targeted inspiratory waveform, shortening inspiratory time will increase peak airway pressure but will not affect overall lung distension if the set tidal volume is unchanged; in fact, higher proximal airway pressures will decrease delivered alveolar volume because of increased compressed volume in the tubing. Conversely, increasing inspiratory time will lower peak airway pressure but could even increase alveolar distension over a series of breaths if insufficient time were provided for complete exhalation, resulting in air-trapping and endogenous positive end-expiratory pressure (auto-PEEP).
It should be remembered that peak inspiratory pressure can also be increased as a result of problems with respiratory system compliance such as low pulmonary parenchymal compliance caused by acute respiratory distress syndrome (ARDS) or pulmonary edema, chest wall problems such as circumferential burns or chest wall edema, and abdominal distension. Such situations will be reflected in an elevated plateau pressure. If this value is high relative to the pressure outside the lung parenchyma—a question that can be sorted out using esophageal manometry37—the transpulmonary pressure difference will be high and the patient may be at increased risk for barotrauma. High plateau pressures in the setting of increased extrapulmonary pressure, on the other hand, will be associated with a small transpulmonary pressure difference and a lower risk of alveolar rupture.
To date, no studies have definitively established that the particular ventilator mode or other ventilator settings such as tidal volume or PEEP affect the incidence of barotrauma. The lack of effect secondary to tidal volume in conventional volume-control ventilation can best be seen by comparing the incidence of barotrauma in the era before and after the widespread adoption of lung-protective ventilation in patients with ARDS. In six large series published between 1974 and 1983, including data from 2980 mechanically ventilated patients, the incidence of detectable extraalveolar air ranged from 4% to 11%, with most in the range of 5% to 7%.28,30,38–41 Although some patients with acute lung injury and ARDS were included in these series, barotrauma was not specifically examined in those conditions. As Tables 44-4 and 44-5 demonstrate, the incidence of barotrauma has not declined significantly since lung-protective ventilation became a part of clinical practice, despite a general impression that this complication is now encountered less frequently than in the past.42
Study (Reference) | Tidal Volume Used in High Tidal Volume Group (N) | Tidal Volume Used in Low Tidal Volume Group (N) | Incidence of Barotrauma in High Tidal Volume Group: N(%) | Incidence of Barotrauma in Low Tidal Volume Group: N(%) |
---|---|---|---|---|
Amato et al44 | 12 mL/kg (24) | ≤6 mL/kg (29) | 10 (42)b | 2 (7) |
Brochard et al45 | 10 to 15 mL/kgb (58) | 6 to 10 mL/kg (58) | 7 (12)c | 8 (14) |
Stewart et al47 | 10 to 15 mL/kga (60) | ≤8 mL/kg (60) | 4 (7)d | 6 (10) |
Brower et al46 | 10 to 12 mL/kga (26) | ≤8 mL/kg (26) | 1 (3.8)e | 2 (7.7) |
ARMA Study43 | 12 mL/kga (432) | <6 mL/kg (429) | 48 (11)e | 43 (10) |
Villar et al48 | 9 to 11 mL/kga (50) | 5 to 8 mL/kg (53) | 4 (8.4)f | 2 (4) |
Total | (650) | (655) | 74 (11.4) | 64 (9.6) |
Study (Reference) | Control Group (N) | Intervention Group (N) | Incidence of Barotrauma in Control Group: N(%) | Incidence of Barotrauma in Intervention Group: N(%) |
---|---|---|---|---|
Brower et al49 | Low PEEP (273) | High PEEP (276) | 27 (10)b | 30 (11) |
Mancebo et al50,a | Supine ventilation (60) | Prone ventilation (76) | 4 (6.7)c | 7 (9.2) |
Meade et al51 | Conventional PEEP; plateau pressure <30 cm H2O (508) | High PEEP; plateau pressure <40 cm H2O (475) | 47 (9.1)c | 53 (11.2) |
Mercat et al52 | PEEP 5 to 9 cm H2O (382) | PEEP set to reach plateau pressure 28 to 30 cm H2O (385) | 22 (5.8)d | 26 (6.8) |
Papazian et al53 | No paralysis (162) | 48 hours of paralysis with cis–atracurium (177) | 19 (11.7)e | 9 (5.1) |
Total | (1385) | (1389) | 119 (8.6) | 125 (9.0) |
In trials comparing low and high tidal-volume strategies,43–48 as well as trials in which both arms were managed with lower tidal volumes,49–53 the incidence of barotrauma does not differ significantly from that documented in earlier periods. Further support for a lack of a role for tidal volume comes from studies that examined risk factors for the incidence of barotrauma, which revealed that tidal volume had no significant effect.47,54,55
It is not clear whether other, less commonly used modes of ventilation affect the incidence of barotrauma. Several studies on the use of high-frequency oscillatory ventilation in acute respiratory failure, for example,56–58 have documented incidence rates of pneumothorax or barotrauma of 8% to 9%, although a retrospective review of high-frequency oscillatory ventilation use at three centers in Toronto documented a much higher incidence rate of 21.8%.59 A randomized trial comparing airway pressure release ventilation to conventional volume-targeted lung-protective ventilation, in patients with acute respiratory failure following trauma, found no difference in the incidence of barotrauma with the two approaches.60
The use of noninvasive ventilation has increased substantially in recent years, particularly for the management of acute exacerbations of chronic obstructive pulmonary disease. One report suggests the incidence of barotrauma is less than 5% with this modality,61 but, overall, less data are available on this question compared to the other support modalities.
By increasing alveolar distension, increased levels of PEEP might be expected to cause more barotrauma. As with tidal volume, however, no studies have established a strong relationship in this regard. Eisner et al62 examined 718 patients enrolled in the ARDS Network clinical trials and found that higher levels of PEEP correlated with increased likelihood of developing clinical barotrauma during the first 4 days (relative hazard: 1.5), although the 95% confidence interval included 1 (0.98 to 2.3). More recently, two large randomized studies49,51 comparing the use of high and low PEEP strategies in patients with ARDS ventilated at low tidal volumes (≤6 mL/kg predicted body weight) showed no significant differences in the incidence of barotrauma. Even if data did suggest an association between PEEP and increased risk of barotrauma, this finding could be interpreted in two ways: either higher levels of PEEP predispose to barotrauma, or sicker patients (who require higher levels of PEEP) are more likely to develop barotrauma.
End-inspiratory plateau pressure is another variable that could affect alveolar distension and, therefore, the risk of barotrauma, but consistent evidence of such a link is also lacking. Boussarsar et al54 reviewed the findings of eleven studies (2270 patients) that reported the incidence of barotrauma in patients with ARDS and noted that end-inspiratory plateau pressure was the only ventilator management-related variable that correlated statistically with the occurrence of barotrauma. In an international study of 5183 mechanically ventilated adult patients with a wide variety of diagnoses, however, Anzueto et al63 found no correlation between any ventilator setting or pressure measurement and the development of barotrauma; several recent large randomized trials of ventilator management in ARDS in which plateau pressures were different between the two arms of the study showed no differences in barotrauma incidence (see Table 44-5).43,51,52 One reason why plateau pressure may not be convincingly related to the risk of barotrauma may be because plateau pressure represents the difference between pressure in the airways and the atmosphere and, hence, total respiratory system compliance, whereas the risk of barotrauma is more a function of the pressure difference between the alveoli and bronchovascular sheath. Plateau pressure may be high but if this is caused by factors outside the lungs, such as increased abdominal distension or chest wall edema, the elevated pressures may not lead to alveolar rupture.
A circumstance in which tidal volumes and airway pressures are delivered substantially above desirable levels is manual (bag) ventilation, an adjunctive ventilation method used immediately following intubation, during cardiopulmonary resuscitation, in association with airway suctioning, and chest physiotherapy, and during patient transport.64 The volumes delivered and the pressures generated are typically not monitored and can vary substantially, depending on the size of the operator’s hands, whether one hand or two is used, and other factors. Tidal volumes during manual ventilation ranged between 838 mL and 1674 mL in one study, with a mean value of 170% of the ventilator’s set tidal volume.65 In a bench study, in which experienced respiratory therapists performed manual ventilation on a lung model to simulate ventilating a 70-kg patient, delivered tidal volumes varied from 400 mL to more than 1000 mL, with airway pressures sometimes exceeding 100 cm H2O.66 Indeed, the development of clinical barotrauma during manual ventilation has been reported several times.67–69
The occurrence of alveolar rupture during mechanical ventilation is influenced markedly by the presence and nature of underlying lung pathology. Barotrauma is rare in patients with normal lungs—for example, in routine postoperative ventilation39 or in paralytic states such as high cervical spinal cord injury or Guillain-Barré syndrome. In contrast, barotrauma is more common in patients with underlying obstructive or restrictive lung disease.42,63 In an observational cohort study of 5183 patients who underwent mechanical ventilation for more than 12 hours in 361 ICUs in twenty countries,70 barotrauma was observed in 154 patients (2.9%).63 The incidence of barotrauma, defined as pulmonary interstitial emphysema, pneumothorax, pneumomediastinum, pneumoperitoneum, or subcutaneous emphysema, was 2.9% in patients with chronic obstructive pulmonary disease, 4.2% in pneumonia, 6.3% in asthma, 6.5% in ARDS, and 10.0% in chronic interstitial lung disease.63 Logistic regression analysis identified the last three of these conditions as independent risk factors for development of barotrauma. An earlier analysis by Gammon et al71 of 168 consecutive patients who underwent mechanical ventilation at one center found with multivariate analysis that of all variables examined, only the presence of ARDS correlated with development of pneumothorax.
Because pressures in the interstitium and bronchovascular sheath tend to be slightly less than those in the alveoli at peak lung inflation, there is a tendency for air to move into these areas once the alveolar walls are disrupted.4–6 Although this is also accepted as the mechanism of the spread of extraalveolar air in spontaneously breathing patients, it is particularly facilitated by positive-pressure ventilation, especially when large tidal volumes are used. Figure 44-2 depicts the possible routes of spread once air leaves the alveolus. Except for systemic air embolism, which is uncommon, and pulmonary interstitial emphysema, the common pathway for all the clinical forms of barotrauma is pneumomediastinum.8 Once in the mediastinum, air follows the path of least resistance and may rupture through the delicate mediastinal fascia and overlying pleura into the pleural space. Why this occurs in some patients and not in others may be determined by local pleural scarring in some cases, but is usually not readily apparent.
Figure 44-2
Pathogenesis of the various forms of barotrauma, with those of greatest clinical importance in bold type. In addition to being associated with use of mechanical ventilation, pneumothorax can also occur as a result of puncture or laceration of the visceral pleura during central line placement or other procedures or traumatic injuries such as rib fractures.
The spread of extraalveolar air to a wide variety of locations in the body can be understood through reference to the fascial planes of the neck, mediastinum, and retroperitoneum, as shown in Figure 44-3.4 Three distinct cervical compartments exist—a previsceral space lying between the deep cervical fascia and the pretracheal fascia, a visceral space lying between the pretracheal fascia and the prevertebral fascia, and a prevertebral space situated behind the prevertebral fascia. These cervical compartments continue via the mediastinum through the thorax and trunk, providing the potential for the spread of air into the retroperitoneal space.
Figure 44-3
Fascial planes of the neck, mediastinum, and retroperitoneum, as depicted at the levels of C-7, T-2, T-5, and L-1, respectively. Because the soft-tissue compartments are continuous, air can dissect into a wide variety of anatomic locations. (Adapted, with permission, from Maunder et al.4) Abbreviations: A, aorta; E, esophagus; inf.V.C., inferior vena cava; P.A., pulmonary artery; Sup.V.C., superior vena cava; T, trachea.
Air may continue to leave the airways, as shown in Figure 44-1, so long as the alveolar rent is large enough and a sufficient pressure gradient exists. If this path of least resistance leads up the mediastinum and into the soft tissues of the neck and upper chest, subcutaneous emphysema may become massive, spreading extensively over the body. Occasionally the pressure gradient and local anatomy favor passage of air into the retroperitoneal space, from which it decompresses into the peritoneum,72–76 causing massive abdominal distension. When the extraalveolar air has reached the pleural cavity, the resultant pneumothorax may rapidly increase with successive positive-pressure breaths. Air readily leaves the torn alveolus and passes into the pleural space, but cannot return between inspirations because of the collapsibility of the lung and mediastinum. A ball-valve mechanism is created and fatal tension pneumothorax can rapidly result. Figure 44-4 depicts examples of the various forms of barotrauma that can result from these mechanisms.
Figure 44-4
Computed tomography image through midchest showing multiple manifestations of pulmonary barotrauma in the same patient including: (A) pneumothorax; (B) pneumomediastinum; (C) pulmonary interstitial emphysema represented by a halo of air surrounding a vascular structure; and (D) subcutaneous emphysema.
After evacuation of a pneumothorax via tube thoracostomy, air may continue to leak into the pleural space and via external suction into the pleural collection device. Such a bronchopleural air leak tends to be perpetuated by the very measures often required to reinflate the collapsed lung and to maintain gas exchange in patients with severe acute respiratory failure. Once a rent exists at either the alveolar or visceral pleural level, the higher the pressure gradient between airways and pleural space, the greater will be the tendency of the bronchopleural fistula to leak. It therefore stands to reason that management should focus on decreasing airway pressure and minimizing pleural suction. As discussed later (see the section Management: Bronchopleural Fistula), however, little experimental evidence is at hand to support this hypothesis, and the management needs of critically ill patients often prevent the realization of these goals.
Clinical Manifestations
The different clinical forms of barotrauma shown in the right-hand column in Figure 44-2 vary considerably in their clinical manifestations, relative frequency, and potential for doing harm to the patient. Table 44-6 lists the nine categories of extraalveolar air that are discussed in the remainder of this chapter, and shows their relative frequency in ventilated patients, along with their relative seriousness and potential threat to life. With few data from prospective studies of the incidence of the different forms of barotrauma across the broad range of patients managed on mechanical ventilation, the frequencies listed in Table 44-6 are based on the our clinical experience and what little information can be gleaned from the literature. Tension pneumothorax and systemic air embolism are always potentially fatal events. Beyond these, however, the effects of barotrauma on survival, organ dysfunction, ICU stay, and other outcomes, separate from those of the underlying disease, are largely unknown.
Form | Relative Frequency | Potential Threat to Life |
---|---|---|
Pulmonary interstitial emphysema | ++++ | + |
Intraparenchymal air cysts | ++ | ++ |
Pneumomediastinum | ++++ | ++ |
Pneumopericardium | + | +++ |
Subcutaneous emphysema | +++ | + |
Pneumoperitoneum | + | ++ |
Systemic air embolism | + | ++++ |
Pneumothorax | +++ | ++++ |
Bronchopleural fistula | ++ | ++ |
Air in the interstitium of the lung can be identified at autopsy in patients who die with clinical barotrauma associated with positive-pressure ventilation, and is easily demonstrated using computed tomography (CT) scanning (see C in Fig. 44-4).77–79 With appropriate technique pulmonary interstitial emphysema may also sometimes be detected on plain radiographs of the chest, and be present without other signs of extraalveolar air.80,81 A variety of patterns have been described.81 Parenchymal stippling, thought to represent multiple small pulmonary vessels and their air-distended vascular sheaths in cross section, has been referred to as a “salt-and-pepper” pattern.80 Air dissection within the pulmonary interstitium is believed responsible for lucent mottling,81 with small cyst-like lucencies that may increase progressively in size on successive films. Other signs of pulmonary interstitial emphysema include lucent streaks, believed to be produced by air dissecting toward the hilum along the course of larger pulmonary vessels, and perivascular halos in the perihilar regions of the lung, representing such air collections seen end-on. All these signs are most readily seen in the presence of diffuse pulmonary consolidation or atelectasis, which increases the radiographic contrast between parenchyma and extraalveolar air.
Although it is well-defined pathophysiologically (see Figs. 44-1 and 44-2), pulmonary interstitial emphysema in mechanically ventilated adult patients has doubtful practical clinical importance, primarily because of the realities of bedside imaging in the ICU. Several older studies described pulmonary interstitial emphysema in critically ill patients,30,31,82–84 and in some instances it has been shown to precede the development of pneumothorax. The subtle changes identifying this entity, however, are beyond the resolution of most bedside radiologic tools. In one study attempting to elucidate the patterns and risk factors for barotrauma in ventilated patients, the investigators abandoned their initial plan to determine whether pulmonary interstitial emphysema precedes pneumothorax because they could not reliably or reproducibly detect the former.85 In our experience, the degree of resolution and interexposure consistency required to detect and follow this radiographic finding are beyond the capabilities of most portable X-ray units, that must frequently shoot through bandages and bedding, with suboptimal positioning and patients who cannot hold still or take a deep breath.
Intrapulmonary cystlike air collections in patients with ARDS have been recognized with increased frequency since the use of CT in such patients became commonplace. The early studies of Maunder et al86 and Gattinoni et al87 demonstrated the heterogeneous nature of lung involvement in ARDS, and especially late in the course of this disorder large collections of free air within the pulmonary parenchyma are common (Fig. 44-5). Pneumothorax also seems to be common in this setting, although no study published to date has documented this observation objectively.
Figure 44-5
CT image through the midchest in a patient with ARDS receiving mechanical ventilation with PEEP. Parenchymal involvement is markedly inhomogeneous, with relatively normal areas in the dependent lung zones, widespread ground glass opacities in both lungs and intraparenchymal cystic air collections, primarily in the anterior portions of the right lung.
Small subpleural air collections, also identified on CT scans occur frequently during the course of ARDS.88 These collections probably represent centrifugal dissection of air through the interstitium to the visceral pleura, and are the likely source for pleural air in some patients.
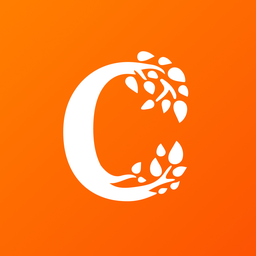
Full access? Get Clinical Tree
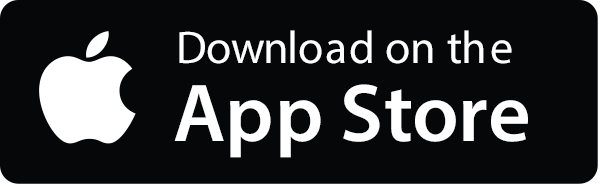
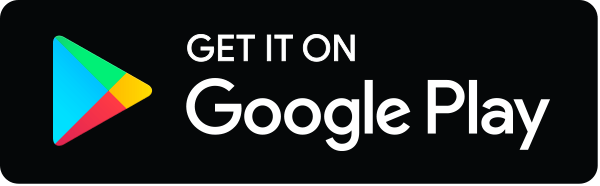