(1)
Division of Pulmonary and Critical Care Medicine, Eastern Virginia Medical School, Norfolk, VA, USA
Keywords
Arterial blood gas analysis (ABG)Hypoxia; hyperoxiaType 1 respiratory failureType II respiratory failureAcidosisAlkalosisMetabolic acidosisRespiratory acidosisPartial pressures of oxygen (PaO2)Partial pressure of carbon dioxide (PaCO2)PhBicarbonate (HCO3 −)Base deficitAlveolar ventilationOxygenationAcid-base balanceHenderson–Hasselbalch equationStrong ion difference (SID)Anion gapOsmolar gapOsmolarityMixed venous oxygen saturation (SmvO2)Metabolic alkalosisEthylene glycolLactic acidosisArterial blood gas (ABG) analysis plays a pivotal role in the management of critically ill patients. Although no randomized controlled study has ever been performed evaluating the benefit of ABG analysis in the ICU, it is likely that this technology stands alone as that diagnostic test which has had the greatest impact on the management of critically ill patients; this has likely been translated into improved outcomes. Prior to the 1960s clinicians were unable to detect hypoxemia until clinical cyanosis developed. ABG analysis became available in the late 1950s when techniques developed by Clark, Stow and coworkers, and Severinghaus and Bradley permitted the measurement of the partial pressures of oxygen (PaO2) and carbon dioxide (PaCO2) [1–3]. The ABG remains the definitive method to diagnose, categorize and quantitate respiratory failure. In addition, ABG analysis is the only clinically applicable method of assessing a patient’s acid-base status. ABGs are the most frequently ordered test in the ICU and have become essential to the management of critically ill patients [4]. Indeed, a defining requirement of an ICU is that a clinical laboratory should be available on a 24-h basis to provide blood gas analysis [5].
Indications for ABG Sampling
ABGs are reported to be the most frequently performed test in the ICU [4]. There are however no published guidelines and few clinical studies which provide guidance as to the indications for ABG sampling [6]. It is likely that many ABGs are performed unnecessarily. Muakkassa and coworkers studied the relationship between the presence of an arterial line and ABG sampling [7]. These authors demonstrated that patients’ with an arterial line had more ABGs drawn than those who did not regardless of the value of the PaO2, PaCO2, APACHE II score or the use of a ventilator. In this study, multivariate analysis demonstrated that the presence of an arterial line was the most powerful predictor of the number of ABGs drawn per patient independent of all other measures of the patient’s clinical status. Roberts and Ostryznuik demonstrated that with use of a protocol they were able to reduce the number of ABGs by 44 % with no negative effects on patient outcomes [4]. The ubiquitous use of pulse oximetry in the ICU has made the need for frequent ABG sampling to monitor arterial oxygenation unnecessary. Furthermore (as discussed below), venous blood gas analysis can be used to estimate arterial pH and bicarbonate (HCO3 −) but not arterial carbon dioxide tension (PaCO2). Previously, ABGs were drawn after every ventilator change and with each step of the weaning process; such an approach is no longer recommended. The indications for ABG analysis should be guided by clinical circumstances. However, as a “general rule” all patients should have an ABG performed on admission to the ICU and/or following (10–15 min) endotracheal intubation. Patients’ with respiratory failure should have an ABG performed at least every 24–48 h. Patients with type II respiratory failure will require more frequent ABG sampling than those with type I respiratory failure. Furthermore, patients with complex acid-base disorders and patients undergoing permissive hypoventilation will require more frequent ABG sampling.
ABG Sampling
ABG specimens may be obtained from an indwelling arterial catheter or by direct arterial puncture using a heparinized 1–5 mL syringe. Indwelling arterial catheters should generally not be placed for the sole purpose of arterial blood gas sampling as they are associated with rare but serious complications. Arterial puncture is usually performed at the radial site. When a radial pulse is not palpable the brachial or femoral arteries are suitable alternatives. Serious complications from arterial puncture are uncommon; the most common include pain and hematoma formation at the puncture site. Laceration of the artery (with bleeding), thrombosis and aneurismal formation are rare but serious complications [8, 9].
ABG analysis is typically performed on whole blood. The partial pressure of oxygen (PaO2,), partial pressure of carbon dioxide (PaCO2), and pH are directly measured with standard electrodes and digital analyzers; oxygen saturation is calculated from standard O2 dissociation curves or may be directly measured with a co-oximeter. The bicarbonate (HCO− 3) concentration is calculated using the Henderson-Hasselbalch equation:
where pKA is the negative logarithm of the dissociation constant of carbonic acid. The base excess is defined as the quantity of strong acid required to titrate blood to pH 7.40 with a PaCO2 of 40 mmHg at 37 °C. In practice, acid is not titrated as suggested but calculated using a variety of established formulae or nomograms. The base excess thus ‘removes’ the respiratory element of acid-base disturbance and identifies the metabolic contribution to interpret with pH and [H+]. The standard bicarbonate is broadly similar and is the calculated [HCO3 −] at a PaCO2 of 40 mmHg. Although the base excess and standard bicarbonate allow for a metabolic acidosis to be diagnosed, it provides few clues as to the pathophysiology or underlying diagnosis.
![$$ \mathrm{p}\mathrm{H}={\mathrm{pK}}_{\mathrm{A}}+ \log \left\{\left[{\mathrm{HCO}}_3^{-}\right]/\left[{\mathrm{CO}}_2\right]\right\} $$](/wp-content/uploads/2016/10/A69851_3_En_22_Chapter_Equa.gif)
As with any diagnostic test it is important that the specimen be collected and processed correctly and that quality assurance methods exist to ensure the accuracy of the measurements. Aside from inter-laboratory variation, errors in calibration and electrode contamination with protein or other fluids may alter results. Heparin is usually added to the blood to prevent coagulation and dilution with older liquid solutions previously caused spuriously low PaCO2. Sample preparation is important because air bubbles falsely elevate PaO2.
The following points must be considered before obtaining sample to avoid errors in blood gas interpretation:
Steady State: Blood sampling must be done during steady state following the initiation or change in oxygen therapy or changes in ventilatory parameters in patients on mechanical ventilation. In most ICU patients a steady state is reached between 3 and 10 min and in about 20–30 min in patients with chronic airways obstruction [10].
Anticoagulants: Excess of heparin may affect the pH. Only 0.05 mL is required to anticoagulate 1 mL of blood.
Delay in processing of the sample: Because blood is a living tissue, O2 is being consumed and CO2 is produced in the blood sample. Red blood cell glycolysis may generate lactic acid and change pH. Significant increases in PaCO2 and decreases in pH occur when samples are stored at room temperature for more than 20 min. In circumstances when a delay in excess of 20 min is anticipated, the sample should be placed in ice; iced samples can be processed up to 2 h without affecting the blood gas values.
Hypothermia. Blood gas values are temperature dependent, and if blood samples are warmed to 37 °C before analysis (as is common in most laboratories), PO2 and PCO2 will be overestimated and pH underestimated in hypothermic patients. The following correction formulas can be used:
Subtract 5 mmHg PO2 per 1 °C that the patient’s temperature is <37 °C
Subtract 2 mmHg PCO2 per 1 °C that the patient’s temperature is <37 °C
Add 0.012 pH units per 1 °C that the patient’s temperature is <37 °C.
ABG Analysis
An ABG provides a rapid and accurate assessment of oxygenation, ventilation, and acid-base status. These three processes are closely interrelated with each other and an alteration in one process will affect the other two. However, for the sake of simplicity and ease of understanding each will be discussed separately.
Alveolar Ventilation
The arterial CO2 content as reflected by arterial CO2 tension (PaCO2) at any given moment depends on the quantity of CO2 produced and its excretion through alveolar ventilation (VA) and can be expressed by the equation, PaCO2 ~ CO2/VA. The alveolar ventilation is that portion of total ventilation that participates in gas exchange with pulmonary blood. If it is assumed that CO2 production is constant, then CO2 homeostasis can be simplified to 1/VA ~ PaCO2. Thus PaCO2 becomes very useful for the assessment of alveolar ventilation. High PaCO2 (>45 mmHg) indicates alveolar hypoventilation and low PaCO2 (<35 mmHg) implies alveolar hyperventilation.
Oxygenation
The ultimate aim of the cardio-respiratory system is to provide adequate delivery of oxygen to the tissues. This is largely dependent upon cardiac output, hemoglobin concentration and hemoglobin saturation. The PaO2 is a measure of the oxygen tension in plasma; while the dissolved fraction makes a negligible contribution to oxygen delivery (<2 %) it is a major factor affecting hemoglobin saturation. In turn the PaO2 is dependent on the concentration of oxygen in the inspired air (FiO2), oxygen exchange in the lung (V/Q mismatching) and the venous oxygen saturation (SmvO2). The PaO2 must always be interpreted in conjunction with the FiO2 and age.
The PaO2 is primarily used for assessment of oxygenation status since PaO2 accurately assesses arterial oxygenation from 30 to 200 mmHg, whereas SaO2 is normally a reliable predictor of PaO2 only in the range of 30–60 mmHg. However, oxygen saturation as measured by pulse oximetry (SpO2) or by ABG analysis (SaO2) is a better indicator of arterial oxygen content than PaO2, since approximately 98 % of oxygen is carried in blood combined with hemoglobin. Hypoxemia is defined as a PaO2 of less than 80 mmHg at sea level in an adult patient breathing room air; the concomitant decrease in cell/tissue oxygen tension is known as hypoxia (or tissue hypoxia). The degree of hypoxia in patients with hypoxemia depends on the severity of the hypoxemia and the ability of the cardiovascular system to compensate. Hypoxia is unlikely in mild hypoxemia (PaO2 = 60–79 mmHg). Moderate hypoxemia (PaO2 = 45–59 mmHg) may be associated with hypoxia in patients with anemia or cardiovascular dysfunction. Hypoxia is almost always (but with a few exceptions) associated with severe hypoxemia (PaO2 <45 mmHg). However, it must be recognized that the human body has an extraordinary capacity to adapt to hypoxemia. Indeed, patients with cyanotic heart disease do not have evidence of tissue hypoxia at rest. Most remarkably, at the balcony of Mount Everest (27,559 ft; 272 Torr) and without supplemental oxygen, experienced mountain climbers have been reported to have a mean PaO2 of 24.6 mmHg in the absence of tissue hypoxia (lactate 2.2 mmol/L) [11]. It would appear that a PaO2 <20 mmHg is unable to sustain life. There is a very steep oxygen diffusion gradient from arterial blood (PaO2 ~ 100) to mixed venous blood (PmvO2 ~ 40 mmHg) to tissue partial pressure PtO2 (10–17 mmHg) to 3–7 mmHg for the cytosolic compartment. Such low values suggest that the oxygen tension at the mitochondria, being at the lowest end of the diffusion pathway which oxygen must travel, is below 5 mmHg. Mitochondria can perform oxidative metabolism at PtO2 as low as 2 mmHg [12, 13].
Relation Between PaO2 and FiO2
The PaO2 alone provides little information regarding the efficiency of oxygen loading into the pulmonary capillary blood. The PaO2 is determined largely by the FiO2 and the degree of intra-pulmonary shunting. The PaO2 must therefore always be interpreted in conjunction with the FiO2. The PaO2 alone does not quantitate the degree of intra-pulmonary shunt, which is required for assessing the severity of the underlying lung disease and in guiding the approach to oxygen therapy and respiratory support. There are various formulas for calculating the intra-pulmonary shunt, including the classic “shunt equation”, which is the gold standard but requires mixed venous sampling through a pulmonary artery catheter, and the alveolar-arterial oxygen gradient equation (see Table 22.1). Clinically the PaO2 to FiO2 ratio (PaO2/FiO2) is commonly used to quantitate the degree of ventilation/perfusion mismatching (V/Q). Since the normal PaO2 in an adult breathing room air with a FiO2 of 0.21 is 80–100 mmHg, the normal value for PaO2/FiO2 is between 400 and 500 mmHg. A PaO2/FiO2 ratio of less than 200 most often indicates a shunt of greater than 20 %. A notable limitation of the PaO2/FiO2 is this it does not take into account changes in PaCO2 at a low FiO2, which tends to have a considerable effect on the ratio.
Table 22.1
Formulas for evaluating patients in respiratory failure
Age-predicted PaO2 = Expected PaO2 − 0.3(age − 25) [expected PaO2 at sea level is 100 mg/Hg] |
As a rough rule of thumb: Expected PaO2 ≈ FiO2 (%) × 5 |
AaDO2 = (FiO2 × [BP* − 47]) − (PaO2 + PaCO2) where BP = barometric pressure |
PaO2/FiO2 ratio |
Oxygenation index = [(Mean airway pressure × FiO2)/PaO2] × 100 |
Vd/Vt = (PaCO2 − PECO2)/PaCO2 (N = 0.2–0.4) |
PaO2 and Age
The normal arterial oxygen tension decreases with age. The normal PaO2 at sea level and breathing room air is approximately 85–90 mmHg at the age of 60 and 80–85 mmHg at the age of 80 years.
Respiratory Failure
Acute respiratory failure occurs when pulmonary system is no longer able to meet the metabolic demands of the body. Respiratory failure is classically divided into type I and type II respiratory failure:
Hypoxemic respiratory failure (type 1)
PaO2 ≤60 mmHg when breathing room air (sea level)
Hypercapnic respiratory failure (type 2)
PaCO2 >= 45 mmHg
Acid-Base Balance
The normal diet generates volatile acid (CO2), primarily from carbohydrate metabolism, and nonvolatile acid (hydrogen ion, H+) from protein metabolism. The aim of the body’s homeostatic system is to maintain pH within a narrow range. pH homeostasis is accomplished through the interaction of the lungs, kidneys and blood buffers. Alveolar ventilation allows for excretion of CO2. The kidneys must reclaim filtered bicarbonate (HCO3 −), because any urinary loss leads to gain of H+. In addition, the kidney must excrete the daily acid load generated from dietary protein intake. Less than half of this acid load is excreted as titratable acids (i.e., phosphoric and sulfuric acids); the remaining acid load is excreted as ammonium. The blood pH is determined by the occurrence of these physiologic processes and by the buffer systems present in the body.
The history of assessing the acid–base equilibrium and associated disorders is intertwined with the evolution of the definition of an acid. In the 1950s clinical chemists combined the Henderson–Hasselbalch equation and the Bronsted–Lowry definition of an acid to produce the current bicarbonate ion centered approach to metabolic acid–base disorders [14]. Stewart repackaged pre-1950 ideas of acid–base in the late 1970s, including the Van Slyke definition of an acid [15]. Stewart also used laws of physical chemistry to produce a “new acid–base” approach [14]. This approach, using the strong ion difference (SID)1 and the concentration of weak acids (particularly albumin), pushes bicarbonate into a minor role as an acid–base indicator rather than as an important mechanism.
The strong ion difference (SID) is not identical to anion gap (AG) and it contains [lactate], although it does share a number of parameters and the trends will often be close. The normal SID has not been well established, although the quoted range is 40–42 mEq/L. As the SID approaches zero, anions ‘accumulate’ and acidity increases. This approach provides a physicochemical model for ‘hyperchloremic acidosis’ following 0.9 % saline administration [21], and the systemic alkalosis of hypoalbuminemia (regarded as a weak acid).
![$$ \mathrm{S}\mathrm{I}\mathrm{D}=\left(\left[{\mathrm{Na}}^{+}\right]+\left[{\mathrm{K}}^{+}\right]+\left[{\mathrm{Ca}}^{2+}\right]+\left[\mathrm{M}{g}^{2+}\right]\right)-\left(\left[{\mathrm{Cl}}^{-}\right]+\left[\mathrm{L} act\mathrm{ate}\right]\right). $$](/wp-content/uploads/2016/10/A69851_3_En_22_Chapter_Equb.gif)
Most clinicians use the bicarbonate ion centered approach for the diagnosis and management of acid-base disorders; this approach is easier to understand and more practical. Furthermore, there is no clinical data to suggest that the Steward approach has any advantages over the classic (bicarbonate) approach [16]. The Steward approach serves to make acid-base interpretation more complex (than it already is) to the point that it confuses rather than simplifies. However, many consider it old fashioned and not “cool” to use the HCO3 − Henderson-Hasselbalch approach. The Henderson-Hasselbalch equation describes the fixed inter-relationship between PaCO2, pH and HCO3 − being described as pH = pK c log HCO 3 −/dissCO 2. If all the constants are removed, the equation can be simplified to pH = HCO3 −/PaCO2 (~Kidney/Lung). The HCO3 − is controlled mainly by the kidney and blood buffers. The lungs control the level of PaCO2 by regulating the level of volatile acid, carbonic acid, in the blood. Buffer systems can act within a fraction of a second to prevent excessive change in pH. Respiratory system takes about 1–15 min and kidneys many minutes to days to readjust H+ ions concentration.
The Anion Gap
Following the principle of electrochemical neutrality, total [cations] must equal total [anions], and so in considering the commonly measured cations and anions and subtracting them, a fixed number should be derived. The measured cations are in excess; mathematically this ‘gap’ is filled with unmeasured anions ensuring electrochemical neutrality. There is never a ‘real’ anion gap, in line with the law of electrochemical neutrality; it is rather an index of non-routinely measured anions. The anion gap is calculated using the following formula [17]:
Critical illness is typically associated with a rapid fall in the plasma albumin concentration. Albumin is an important contributor of the “normal” anion gap. Therefore, as the albumin concentration falls it tends to reduce the size of the anion gap, or have an alkalinizing effect. Various corrections are available, however, Figge’s AG correction (AGcorr) is most commonly used [17]:
![$$ \mathrm{Anion}\;\mathrm{Gap}=\left[\mathrm{N}\mathrm{a}\right]-\left(\left[\mathrm{C}\mathrm{l}\right]+\left[{\mathrm{HCO}}_3^{-}\right]\right):\mathrm{Normal}\;10\pm 2\;\mathrm{m}\mathrm{e}\mathrm{q}/\mathrm{L} $$](/wp-content/uploads/2016/10/A69851_3_En_22_Chapter_Equc.gif)
Albumin gap = 40 − Apparent albumin (normal albumin = 40 g l).
AGcorr = AG + (Albumin gap/4).
A Step Wise Approach to Acid-Base Disorders
Step 1. Do a comprehensive history and physical exam
A comprehensive history and physical examination can often give clues as to the underlying acid-base disorder (see Table 22.2). For example, patients who present with gastroenteritis manifested as diarrhea typically have a non-anion gap metabolic acidosis from loss of fluid containing HCO3 −. Patients who present with chronic obstructive lung disease usually have underlying chronic respiratory acidosis from retention of CO2.
Table 22.2
Common clinical states and associated acid-base disorders
Clinical state | Acid-base disorder |
---|---|
Pulmonary embolus | Respiratory alkalosis |
Hypotension/shock | Metabolic acidosis |
Severe sepsis | Metabolic acidosis, respiratory alkalosis |
Vomiting | Metabolic alkalosis |
Severe diarrhea | Metabolic acidosis |
Renal failure | Metabolic acidosis |
Cirrhosis | Respiratory alkalosis |
Pregnancy | Respiratory alkalosis |
Diuretic use | Metabolic alkalosis |
COPD | Respiratory acidosis |
Diabetic keto-acidosis | Metabolic acidosis |
Ethylene glycol poisoning | Metabolic acidosis |
Post Normal Saline resuscitation | Metabolic acidosis (non-anion gap) |
Step 2. Order simultaneous arterial blood gas measurement and chemistry profile
Step 3. Check the consistency and validly of the results. Normal ABG results are provided in Table 22.3 .
Table 22.3
Normal Acid-Base values
Mean | 1 SD | 2 SD | |
---|---|---|---|
PaCO2 (mmHg) | 40 | 38–42 | 35–45 |
pH | 7.4 | 7.38–7.42 | 7.35–7.45 |
HCO3 (meq/L) | 24 | 23–25 | 22–26 |
Step 4. Identify the primary disturbance
The next step is to determine whether the patient is acidemic (pH < 7.35) or alkalemic (pH > 7.45) and whether the primary process is metabolic (initiated by change in HCO3 −) or respiratory (initiated by a change in PaCO2) See Table 22.4 .
Table 22.4
Acid Base disorders
Acid-base disorder | Criteria |
---|---|
Respiratory acidosis | > 45 mmHg |
Respiratory alkalosis | PaCO2 <35 mmHg |
Acute respiratory failure | PaCO2 >45 mmHg; pH <7.35 |
Chronic respiratory failure | PaCO2 >45 mmHg; pH 7.36–7.44 |
Acute respiratory alkalosis | PaCO2 <35 mmHg; pH >7.45 |
Chronic respiratory alkalosis | PaCO2 < 35 mmHg; pH 7.36–7.44 |
Acidemia | pH < 7.35 |
Alkalemia | pH > 7.45 |
Acidosis | HCO3 < 22 meq/L |
Alkalosis | HCO3 > 26 meq/L |
Step 5. Calculate the expected compensation
Any alteration in acid-base equilibrium sets into motion a compensatory response by either the lungs or the kidneys. The compensatory response attempts to return the ratio between PaCO2 and HCO3 − to normal and thereby normalize the pH. Compensation is predictable; the adaptive responses for the simple acid-base disorders have been quantified experimentally [18] (see Table 22.5). Determine whether the compensatory response is of the magnitude expected i.e. is there a secondary (uncompensated) acid-base disturbance.
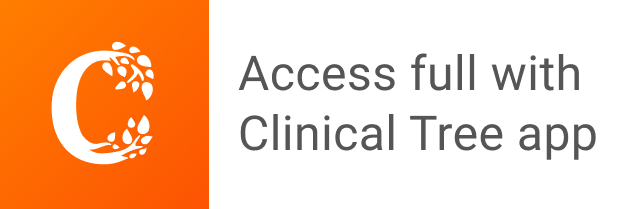