CHAPTER 97 ANTIBACTERIAL THERAPY: THE OLD, THE NEW, AND THE FUTURE
Infections remain the leading cause of death in hospitalized patients, and antimicrobial therapy is a mainstay of treatment. However, widespread overuse and misuse of antibiotics have led to an alarming increase in multiple-drug-resistant (MDR) pathogens. New agents may allow shorter courses of therapy and prophylaxis, which are desirable for cost control and control of microbial flora. Moreover, antibiotics are second only to analgesic agents in the number of adverse drug reactions.
PRINCIPLES OF PHARMACOKINETICS
Half-life refers to the amount of time required for the drug concentration to reduce by half, and thus is a hybrid of consider ations of both clearance and volume of distribution. Half-life is useful to estimate when a steady-state drug concentration will be achieved. If a “loading dose” is not administered intravenously, thereby creating instantaneously a desired drug concentration to be maintained throughout therapy, four to five half-lives must elapse to achieve a steady state. Changes in dosage and changes in half-life owing to disease state (e.g., renal failure) must be accounted for. Interpretation of drug concentration data is difficult if the patient is not at a steady state, especially so in critical illness characterized by fluctuating organ function and volume of distribution.
EMPIRIC ANTIBIOTIC THERAPY
Must antibiotics be started immediately? If the presumed infection is not destabilizing, this decision also depends on the overall status of the patient and should take into consideration such host factors as age, debility, renal and hepatic function, and immunosuppression. Culture yields are highest before antibiotics are administered, which for certain types of specimens (e.g., blood, cerebrospinal fluid) can be crucial. However, for many infections (e.g., bacteremia, intraabdominal infection, pneumonia) early appropriate therapy improves outcome.
CHOICE OF ANTIBIOTIC
The choice of which antibiotic to prescribe is made based on several interrelated factors. Paramount is activity against identified pathogens, presuming that a distinction between infecting and colonizing organisms can be made and that narrow-spectrum coverage is always most desirable. Knowledge of antimicrobial resistance patterns, nationally and especially in one’s own institution and unit, is essential. Also important is an assumption regarding likely pathogens, which is paramount in cases where empiric therapy is necessary. Estimation of likely pathogens depends on the disease process believed responsible, whether the infection is community- or hospital-acquired, whether MDR organisms are present, and proximity to other infected patients. Also important are patient-specific factors, including age, debility, immunosuppression, intrinsic organ function, prior allergy or another adverse reaction, and recent antibiotic therapy. Institutional factors that may play a role include the existence of guidelines or practice parameters that may specify a particular therapy, or the availability of specific agents as defined by inclusion on the formulary or restriction by antibiotic control programs (Figure 1).
Development of Bacterial Resistance
Cephalosporin resistance among Gram-negative bacilli can be the result of induction of chromosomal β-lactamases after exposure to the antibiotic. The extended-spectrum cephalosporins are rendered ineffective when bacteria such as enteric Gram-negative bacilli mutate to constitutively produce a β-lactamase that is normally an inducible enzyme. Although resistance to cephalosporins can occur by several mechanisms, the appearance of chromosomally mediated β-lactamases has been identified as a consequence of the use of third-generation cephalosporins. Resistance rates decline when use is restricted. The induction of an extended-spectrum β-lactamase (ESBL) in Klebsiella by ceftazidime was first reported approximately 20 years ago, but more than 200 mutations have now been described in several species of Gram-negative bacteria. The mutant bacteria develop resistance rapidly not only to all cephalosporins but to entire other classes of β-lactam antibiotics. It is therefore justifiable to restrict the use of ceftazidime, especially in institutions grappling with an ESBL-producing bacterium. The carbapenems generally retain useful microbicidal activity against ESBL-producing strains. Increasingly, Pseudomonas aeruginosa produces beta-lactamases of the ampC type.
ANTIBIOTIC SPECTRUM OF ACTIVITY
Penicillins
The carboxypenicillins (ticarcillin and carbenicillin) and ureidopenicillins (azlocillin, mezlocillin, and piperacillin; sometimes referred to as acylampicillins) have enhanced activity against Gram-negative bacteria and some activity against P. aeruginosa. Ureidopenicillins have greater intrinsic activity against Pseudomonas, but with the advent of β-lactamase inhibitor combination drugs none of these agents is used widely anymore. Beta-lactamase inhibitors (sulbactam, tazobactam, and clavulanic acid) result in enzymatic inactivation and enhanced effectiveness of the antibacterial agent. The effectiveness of these drugs as antibacterial agents is primarily a function of the inherent antibacterial properties of the parent compound (ampicillin < ticarcillin < piperacillin), and to a lesser extent of the effectiveness of the inhibitor (sulbactam ∼ clavulanic acid < tazobactam). The spectrum of activity varies as a result, and the treating clinician needs to be familiar with each of the drugs in this class.
< div class='tao-gold-member'>
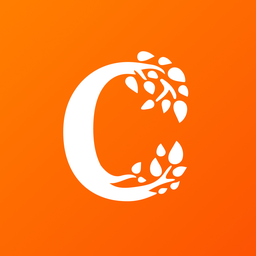
Full access? Get Clinical Tree
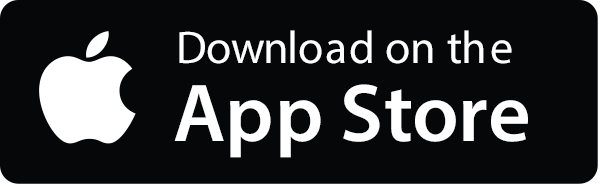
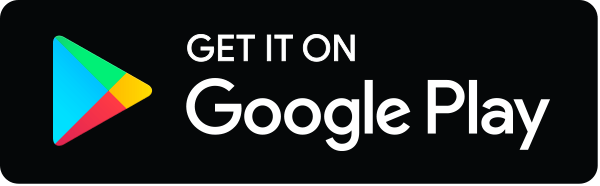