1
The decrease in metabolism seen with the volatile anesthetics is due to a reduction in organized neurotransmission, reaching a maximum reduction in CMRO2 of approximately 50% when an isoelectric electroencephalogram (EEG) is achieved (Fig. 2.1). The remaining 50% of the cerebral metabolic requirement represents the energy expended in maintaining cellular integrity, and is not amenable to reduction by administration of anesthetic agents. Burst suppression, periods of isoelectric EEG interrupted by brief bursts of electrical activity, is a pattern which is seen during the transition from continuously active to continuously isoelectric EEG waveforms, and is associated with a reduction in cerebral energy requirement similar to that obtained with a completely isoelectric EEG (Fig. 2.2). This is a clinically useful target for metabolic suppression during periods of anticipated ischemia, because no additional benefit has been shown with deeper levels of anesthesia. In addition, anesthesia doses beyond those required for burst suppression increase the risk of both delayed emergence and cardiovascular collapse.
CLINICAL PEARL
There is no evidence for improved outcomes with deeper anesthesia once burst suppression is achieved.
FIGURE 2.1 Cerebral metabolic rate for oxygen decreases with increasing effect-site concentration of anesthetic, to a maximum reduction of 50% of baseline. At this point an isoelectric EEG is observed. (Adapted from Newfield P, Cottrell J. Handbook of Neuroanesthesia: Clinical and Physiologic Essentials. 1st ed. Boston, MA: Little, Brown and Co.; 1983:21.)
FIGURE 2.2 Representative EEG waveforms for awake, anesthetized, and burst-suppressed states. Note the periodic electrocardiographic activity visible on the burst-suppressed EEG tracing.
At high concentrations, intracranial pressure (ICP) may increase due to increases in CBF and cerebral hyperemia. However, the increase in CBF that occurs secondary to the loss of cerebral autoregulation at high anesthetic concentrations (Fig. 2.3), may be limited by a decrease in cerebral perfusion pressure (CPP) due to a reduction in the mean arterial pressure (MAP) from both systemic vasodilation and myocardial depression. In addition, since the cerebrovascular response to CO2 is generally maintained, hyperventilation may offset any increase in CBF, CBV, and thus ICP.
FIGURE 2.3 Attenuation of cerebral autoregulatory response by inhaled anesthetic gases. At greater than 1.5 MAC, cerebral blood flow is directly related to cerebral perfusion pressure. (Adapted from Newfield P, Cottrell J. Handbook of Neuroanesthesia: Clinical and Physiologic Essentials. 1st ed. Boston, MA: Little, Brown and Co.; 1983:18.)
In addition to these direct effects on CMRO2 and blood flow, the volatile agents also have variable effects on cerebrospinal fluid production and reabsorption, which independently impact ICP, CPP, and ultimately CBF. These effects, however, are slow to develop (with timeframes on the order of several hours), and in practice may be of limited clinical importance.
1. Sevoflurane. At concentrations less than one-half the minimum alveolar concentration (MAC), sevoflurane minimally disrupts cerebral flow-metabolism coupling and autoregulation [1]. With increasing concentration progressive decreases in CMRO2 occur, with burst suppression obtained at concentrations of 1.5 to 2 MAC. At the same time, concentrations greater than 0.5 MAC cause dose-dependent cerebral vasodilation and a resulting increase in CBF, weakening the coupling of flow to metabolism [2,3]. Autoregulation and the reactivity to arterial carbon dioxide concentrations are maintained, and hyperventilation will attenuate the increases in blood flow [1,4].
Some observers have reported epileptiform changes in the EEG during anesthesia with sevoflurane, most notably during inhalational induction and about the time burst suppression is achieved [5]. Reported activity has ranged from periodic spike and wave discharges to (rarely) frank seizure activity. While seizure activity is clearly associated with increases in CMRO2 and blood flow, the clinical significance of the more often reported periodic discharges is unknown.
Sevoflurane decreases both CSF production and reabsorption in parallel, with uncertain effects on overall CSF balance and ICP [6].
2. Isoflurane. The general nature of isoflurane’s effect on CBF and metabolism is similar to that of sevoflurane, with maximal depression of CMRO2 and increases in CBF achieved at approximately 2 MAC. For a given fraction of its respective MAC, isoflurane produces less cerebral vasodilation but greater metabolic depression than sevoflurane, making it the preferred volatile anesthetic for neuroanesthesia in some circles [2,7]. Cerebrovascular autoregulation is well maintained at concentrations less than 0.5 MAC, and may be restored at higher concentrations when combined with moderate hypocapnia [8].
Isoflurane has no effect on CSF production and a bimodal effect on CSF reabsorption, with impaired reabsorption at low concentrations and increased reabsorption at high concentrations. The net effect promotes increased ICP at low isoflurane concentrations and decreasing ICP at high concentrations [9].
3. Desflurane. As compared with sevoflurane and isoflurane, desflurane produces similar cerebral vasodilation and depression of CMRO2 [10], with clinically relevant effects over a range of approximately 0.5 to 2 MAC. Desflurane does, however, have slightly greater vasodilatory potency as compared with the other volatile anesthetics in common use today [7,11]. In addition, rapid increases in desflurane concentration increase sympathetic outflow, potentially compounding the anesthetic’s effect in increasing CBF by elevating systemic blood pressure. Cerebral vascular response to CO2 remains intact to at least 1 MAC of desflurane [12].
Desflurane has little effect on CSF production or reabsorption, except in the clinically relevant setting of combined hypocapnia and intracranial hypertension [13]. In one relevant study, under those conditions desflurane increases CSF production. This has the unfortunate consequence of promoting further increases in ICP under the worst possible clinical circumstances.
CLINICAL PEARL
The cerebrovascular and cerebral metabolic effects of the potent inhalational anesthetics are similar, with minor variations on specific parameters.
B. Nitrous oxide. In contrast to the volatile inhaled anesthetics, nitrous oxide causes increases in CMRO2, CBF, and ICP [14]. Increases in CBF exceed the increases in CMRO2 with a nitrous oxide anesthetic, resulting in decoupling of flow from metabolism despite increases in both parameters. This effect can be attenuated by the addition of intravenous agents, including propofol, barbiturates, and opioids. Sevoflurane, on the other hand, is synergistic with nitrous oxide in increasing CBF and therefore further weakens flow-metabolism coupling. Interestingly, when cerebral electrical silence is induced with propofol, the addition of nitrous oxide may restore EEG activity [15].
2
Cerebrovascular reactivity to CO2 is maintained during nitrous oxide anesthesia [16], and CSF homeostasis is unaltered.
C. Xenon. Although its use as a general anesthetic is currently limited due to scarcity and expense, xenon exhibits several favorable properties for use in neuroanesthesia. Xenon exhibits neuroprotective properties, possibly through antagonism of the N-Methyl-D-Aspartate (NMDA) receptor, which plays a role in excitotoxic cellular demise. Xenon is also noted for exceptional cardiovascular stability, and cerebrovascular autoregulation and CO2 reactivity both appear to be maintained under xenon anesthesia at 1 MAC [17]. CBF exhibits regional variations consistent with regional variations in metabolism, with a global trend toward reductions in both, CMRO2 more so than CBF [18–20]. The effects of xenon on CSF production and reabsorption are unknown.
D. Carbon dioxide. As discussed in the previous chapter and repeatedly referenced here, arterial carbon dioxide tension correlates strongly and in an inverse direction with cerebral vasomotor tone in the normal physiologic condition. This correlation is preserved under all but the most extreme physiologic derangements, both iatrogenic and pathologic.
Moderate hypercapnia is sedating, and reduces CMRO2 in a dose-dependent fashion for a PaCO2 of up to at least 70 mm Hg [21,22]. With higher arterial carbon dioxide tension, sympathetic activation is observed, though how this correlates with cerebral metabolic activity is unknown.
II. Intravenous anesthetics
A. Induction agents. With the exception of ketamine, intravenous induction agents uniformly generate reductions in CBF and CMRO2, with maintenance of flow-metabolism coupling (Table 2.2). In addition to induction of anesthesia, these agents are often used during maintenance of anesthesia to supplement inhalational anesthetics, in order to provide burst suppression for brain protection, or as part of a total intravenous anesthetic (TIVA) to better accommodate neurophysiologic monitoring.
Table 2.2 Effects of intravenous anesthetics on cerebral hemodynamics
3
1. Propofol. Propofol produces dose-dependent reductions in CBF, CMRO2, and thus ICP [14], to the point of abolishment of cortical electrical activity. Flow-metabolism coupling is maintained [23], with the caveat that global cardiovascular depression associated with propofol administration may reduce CPP below the lower limits of autoregulatory compensation. Vasopressor support may be required to maintain cerebral perfusion when propofol is used to induce EEG burst suppression. CO2 reactivity is maintained, and propofol has no effect on the production or reabsorption of CSF [24].
2. Barbiturates. Thiopental is the archetype for intravenous anesthetics used for metabolic suppression, and like propofol the barbiturates produce dose-dependent reductions in CMRO2, CBF, and ICP, up to the point of EEG isoelectricity [25–27]. Flow-metabolism coupling and CO2 reactivity are also maintained with these agents. High-dose barbiturates may require concomitant vasopressor support to maintain CPP, similar to propofol.
It should be noted that while the barbiturate methohexital does induce reductions in CBF and CMRO2 in a dose-dependent fashion similar to the other barbiturates, this medication has been shown to lower the seizure threshold [28,29]. As a result, seizure activity on emergence from general anesthesia with methohexital may be a concern. When it occurs, the increased metabolism associated with seizure activity far exceeds any erstwhile reductions in CMRO2, and it is accompanied by large increases in CBF as a result.
3. Etomidate. Etomidate also produces dose-dependent reductions in CMRO2, CBF, and ICP with the capacity to produce an isoelectric EEG [30,31]. Flow-metabolism coupling, autoregulation, and CO2 reactivity are all maintained under etomidate anesthesia. Etomidate has the useful characteristic of causing less cardiovascular depression than either propofol or barbiturates; however, the fact that it causes clinically significant adrenocortical suppression has led to a decline in its use. There is also evidence that regional tissue oxygenation in the brain may decrease under burst suppression induced with etomidate [32,33], a problematic finding in the setting of neurosurgical interventions.
Etomidate is especially useful in the setting of neurophysiologic monitoring for its ability to maintain both somatosensory evoked potential (SSEP) signal amplitude and transcranial motor evoked potential (TcMEP) thresholds [34,35].
At low doses etomidate has no effect on CSF homeostasis, while at high doses it causes a decrease in production [36], promoting a reduction in ICP.
2
4. Ketamine. Ketamine produces increases in both CBF and CMRO2 in spontaneously breathing patients, with a disruption in flow-metabolism coupling such that the increase in CBF exceeds that of CMRO2 [37]. These effects are analogous to those seen with nitrous oxide. Autoregulation and CO2 reactivity are preserved.
The increase in CBF seen with ketamine may be due to a combination of hypoventilation and hypercarbia in spontaneously ventilated patients, direct cerebral vasodilation, and increased CPP due to sympathomimetic activity [37]. Ketamine is also an NMDA receptor antagonist that has been shown to be neuroprotective in the face of cerebral ischemia.
Historically, induction doses of ketamine have been thought to significantly increase ICP, presumably due to the aforementioned effects, and the drug has been considered to be contraindicated in patients with increased ICP. Other studies suggest that ketamine may be safe for the patient with increased intracranial elastance so long as moderate hypocarbia is maintained [38–40], but the debate is still open and prudence would suggest the avoidance of induction doses of ketamine for patients with increased intracranial elastance (increased changes in pressure in response to changes in volume) under most circumstances.
In patients who are sedated or anesthetized and mildly hyperventilated, however, the preponderance of evidence suggests that ketamine does not increase and in fact may lead to a reduction in ICP.
Like etomidate, ketamine has also been found to be clinically useful during neurophysiologic monitoring to preserve SSEP signal amplitude and TcMEP thresholds under anesthesia [35,41,42].
Ketamine also decreases reabsorption of CSF [43], and this is an additional mechanism by which it may increase ICP in some cases.
CLINICAL PEARL
There is greater heterogeneity in cerebral vascular and metabolic effects amongst intravenous anesthetics than there is between inhalational anesthetics.
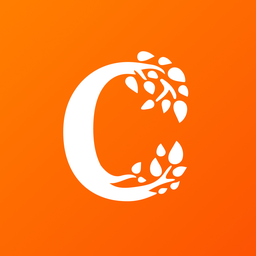
Full access? Get Clinical Tree
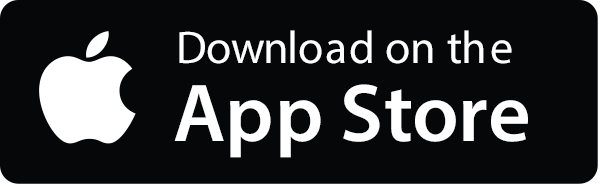
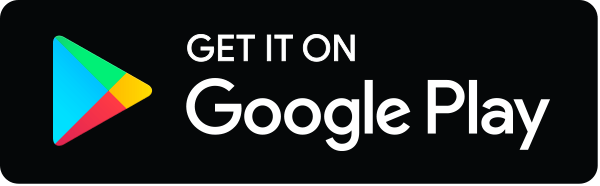